INTRODUCTION
Ketosis is common metabolic disease of dairy animals, and is associated with high milk yield during lactation or inadequate feed intake for energy that results in negative energy balance (NEB) [1]. Ketosis in lactating dairy cow decreases milk production and has negative effects on reproductive capacity [2]. In addition, such cows have a higher risk of developing periparturient disease such as lameness, mastitis, metritis, and retained placenta [3–6]. Ketosis is associated with an increased concentration of ketone bodies (acetoacetate, acetone and beta-hydroxybutyrate [BHB]) in the biofluid (milk, plasma, serum and urine) [7]. The underlying cause of the high concentration of ketone body metabolites are low blood glucose levels associated with hypoinsulinemia, which results in the mobilization of fatty acid (FA)s generated from adipose tissue [7]. Ketosis is classified into subclinical ketosis (SCK), clinical ketosis (CK), and type I and II ketosis. In SCK and CK, BHB concentration ranges from 1.2 to 1.4 mM/L and 2.6 to 3.0 mM/L, respectively [8,9]. Type I ketosis occurs between 3 and 6 weeks postpartum when the energy requirement for milk production is the highest [10]. Type II ketosis can lead to complications such as fatty liver via an increase in blood glucose and insulin levels because of excessive feed intake during the dry period [7,10]. Ketosis leads to considerable economic loss in the dairy industry [11]; therefore, research on the prevention and diagnosis of ketosis is required.
Metabolomics studies pertaining to ruminant biofluids including milk, plasma, rumen fluid, serum and urine are performed using many metabolite analyzers (nuclear magnetic resonance [NMR] spectroscopy, gas chromatography-mass spectrometry [GC-MS], liquid chromatography-mass spectrometry [LC-MS], etc.). Moreover, metabolomics studies pertaining to metabolic diseases (acidosis, ketosis etc.) have been conducted. Ketosis research using metabolites in the serum and plasma and the comparative study of metabolites in the plasma of CK, SCK and healthy lactating dairy cows have identified several metabolic pathways associated with CK and SCK by GC-MS, NMR and LC-MS [11–13]. In another study, plasma metabolite profiling was performed for type I and II ketosis by proton NMR (1H-NMR) spectroscopy [14]. Another study compared the urine metabolites in healthy and SCK cows by NMR, direct injection GC-MS and LC-MS/MS [15]. However, there have been fewer urine-based research on ketosis in lactating dairy cows compared with those on other biofluids such as serum, plasma and milk. Metabolic profiling analysis of urine samples has identified biomarkers in various studies on humans [16,17]. Zhang et al. [15] reported a lacked of urinary metabolomics profiling for the identification of predictive biomarkers of ketosis in dairy cows. Therefore, it is necessary to study the urine metabolites of ketosis-induced dairy cows. Such studies will be useful in the future to search for biomarker candidates for ketosis diagnosis using blood (serum and plasma) and urine.
Recently, a metabolomics study on Hanwoo cattle and Holstein cows biofluids was performed by 1H-NMR spectroscopy in Korea [18–20]. However, there have been few metabolomics studies on lactating dairy cow biofluids associated with ketosis Korea compared with those in other countries. In addition, in the Korea, the Holstein species are representing lactating dairy cow, and most important ruminant breeds in the Korean dairy industry [21]. Therefore, it is essential to conduct metabolomics studies that can help us to identify metabolic biomarkers biofluids that can diagnosis ketosis in the future.
We hypothesized that the serum and urine metabolites profiling of lactating dairy cows would be different between healthy (CON) and SCK groups. To test this hypothesis, we aimed to investigate the serum and urine metabolites profiling of lactating dairy cows using 1H-NMR spectroscopy and compare between two groups. This metabolic study will be helpful for developing strategies to reduce lactating dairy cow ketosis in Korea.
MATERIALS AND METHODS
CON and SCK Holstein lactating dairy cow general information (month-old, body weight, parity, milk yield, BHB concentration and number of experimental animals), total experimental day (14 days), diet adaptation period (4 days) blood BHB concentration monitoring period (9 days), blood and urine samples collected day (last day of the experiment) were same of previously Eom et al. study [22].
All experimental cows were fed total mixed ration (TMR). The consumed CON and SCK groups feed intake amount, same of previously Eom et al. study [22]. The results of chemical composition of the TMR is shown Table 1. Contents of acid detergent fiber, calcium, crude protein, dry matter, neutral detergent fiber and phosphorus in TMR was determined as described by Association of Official Analytical Communities [23,24] and Van Soest et al. [25] methods.
Zin Care, Contained 16 GDU/g protease bromelain, 2.0 × 108 cfu/g. Supex-F, Contained 99% protected fat from of palm oil (Cofavet, Cheonan, Korea).
Trace minerals, Contained 0.40% Mg; 0.20% K; 4.00% S; 0.08% Na; 0.03% Cl; 400 mg of Fe/kg; 60,042 mg of Zn/kg; 16,125 mg of Cu/kg; 42,375 mg of Mn/kg.
Blood samples collected and storage methods until 1H-NMR spectroscopy analysis was determined as described by Kim et al. [19] and Eom et al. [20] methods. Urine samples collected and storage methods until 1H-NMR spectroscopy analysis was determined as described by Kim et al. [19] and Eom et al. [20] methods.
The 1H-NMR spectroscopy analysis of serum and urine samples was performed by following methods modified from Sun et al. [26], Jung et al. [27], Bertram et al. [28] and Jeong et al. [29] methods.
The spectra of two samples were obtained on a SPE-800 MHz NMR-MS Spectrometer (Bruker BioSpin AG, Fällanden, Switzerland) at 298 K using a 5 mm triple-resonance inverse cryoprobe with Z-gradients (Bruker BioSpin, Billerica, MA, USA) and method condition described by Kim et al. method [30].
Serum and urine metabolites identification and quantification methods and data collected was performed by following methods modified from Kim et al. [19] and Eom et al. [20] methods. Metabolites data statistical analyses were using the Metaboanalyst version 5.0 program (https://www.metaboanalyst.ca), an open source R-based program for metabolomics. For the serum and urine metabolites analysis, when 50% of samples were under the identification limit or had at least 50% of missing values, they were eliminated from the analysis. The missing values were replaced by a value one-half of the minimum positive value from the original data. In addition, statistical analyses methods were determined as described by Kim et al. [19] and Eom et al. [20] methods. Univariate Student’s t-test were used to quantify difference between metabolite profiles of the serum and urine samples. In addition, principal component analysis (PCA), partial least square-discriminant analysis (PLS-DA), variable importance in projection (VIP) scores and metabolic pathway results were determined as described by Kim et al. [19] and Eom et al. [20] methods.
RESULTS
To analyze the variations in the serum and urine metabolites profiling of CON and SCK groups, we were performed PCA and PLS-DA. In the serum PCA score plot (Fig. 1A), two groups were not separated (PC 1 : 30.2%; PC 2 : 24%). In the urine PCA score plots (Fig. 1B) two groups were not separated (PC 1 : 31%; PC 2 : 24.5%).
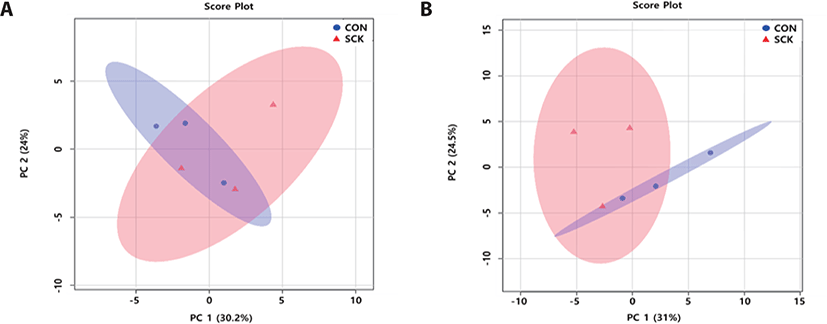
The serum PLS-DA score plots (Fig. 2A), for the two groups were clearly separated (component 1 : 24.6%; component 2 : 21.6%). The urine PLS-DA score plots (Fig. 2B) for two groups were clearly separated (component 1 : 28.1%; component 2 : 23.3%). These results show different in the concentration of serum and urine metabolites between CON and SCK groups.
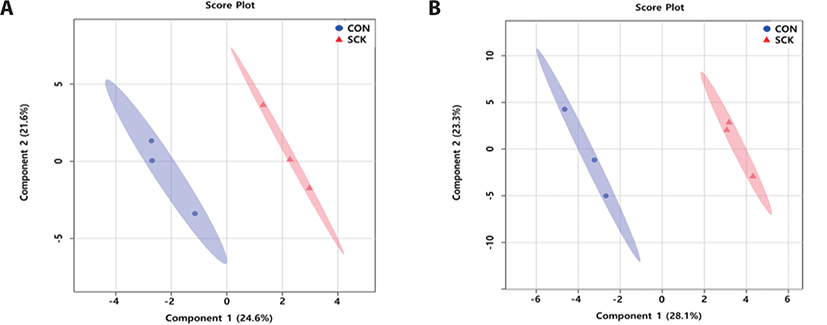
Supplementary Tables S1–S7 and, Supplementary Figs. 1 and 2 summarize the detected and quantified metabolites in the two groups. In the CON group, 98 metabolites were detected and divided into 12 chemical classes in the serum. In addition, a total of 52 metabolites were quantified. In the SCK group, 83 metabolites were detected and divided into 12 chemical classes in the serum. In addition, a total of 55 metabolites were quantified.
In the CON group, 144 metabolites were detected and divided into 13 chemical classes in the urine. In addition, a total of 93 metabolites were quantified. In the SCK group, 168 metabolites were detected and divided into 14 chemical classes in the urine. In addition, a total of 93 metabolites were quantified.
Table 2 shows the significant trends (p < 0.05) and tendencies (0.05 ≤ p < 0.1) of different metabolites in the serum and urine of the two groups. Acetoacetate and succinate levels were significantly higher, whereas acetate, galactose and pyruvate levels tended to be higher but non-significantly in the serum of the SCK group than in the CON group. In contrast, 5-aminolevulinate (5-ALA) and betaine levels were significantly higher, whereas lactulose and 3-methylxanthine (3-MX) levels tended to be higher in the CON group than in the SCK group.
Variable importance in the projection obtained from partial least square-discriminant analysis model.
Indole-3-acetate, theophylline, p-cresol, 3-hydroxymandelate, gentisate, N-acetylglucosamine, N-nitrosodimethylamine, xanthine and pyridoxine levels in the urine of the SCK group were significantly higher, and nicotinurate, acetoin, trimethylamine N-oxide, 3-MX, indole-3-lactate, carnosine and BHB levels tended to be higher compared with those in the CON group. In contrast, homogentisate, ribose, gluconate, ethylene glycol, maltose, 3-methyl-2-oxovalerate and glycocholate levels were significantly higher, and alanine level tended to be higher in the CON group than in the SCK group.
As shown in Figs. 3A and 3B, the evaluation of over to 1.5 VIP score of PLS-DA model showed 15 and 18 metabolites between the two groups of serum and urine, respectively. Acetoacetate, pyruvate and O-acetylcholine were higher VIP scores in the serum of the SCK group compared with those in the CON group. In contrast, 3-MX, syrinagate and arginine were higher VIP scores in the serum of the CON group compared with those in the SCK group. Theophylline, gentisate and N-nitrosodimethylamine were higher VIP scores in the urine of the SCK group compared with those in the CON group. In contrast, ethylene glycol, gluconate and maltose were higher VIP scores in the urine of the CON group compared with those in the SCK group.
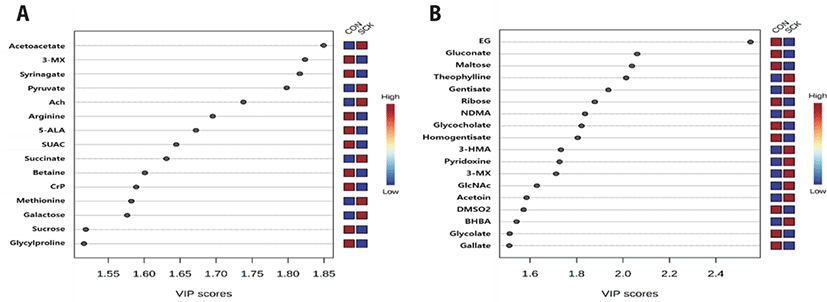
In the serum profiling of including porphyrin and chlorophyll metabolism; glycine, serine and threonine metabolism; citrate cycle (tricarboxylic acid [TCA] cycle); and alanine, aspartate, and glutamate metabolism, four metabolic pathways significantly (p < 0.05) differed between the two groups. The following four metabolic pathways tendency (0.05 ≤ p < 0.1) differed in the serum between the two groups; such as tyrosine metabolism; butanoate metabolism; synthesis and degradation of ketone bodies; and valine, leucine and isoleucine degradation (Table 3 and Fig. 4A).
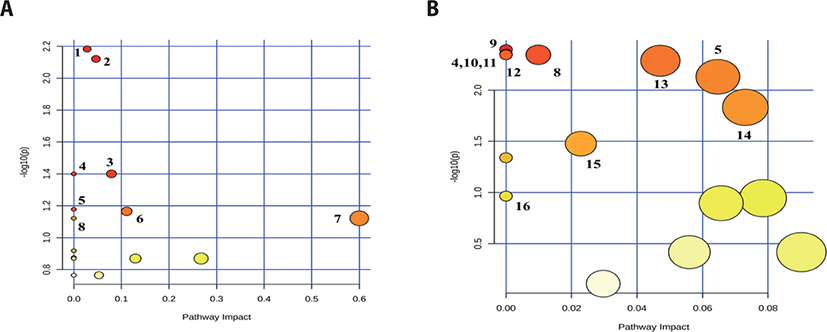
In the urine, the following 11 metabolic pathways significantly (p < 0.05) differed between the two groups; such as ubiquinone and other terpenoid-quinone biosynthesis; alanine, aspartate and glutamate metabolism; selenocompound metabolism; aminoacyl-tRNA biosynthesis; valine, leucine and isoleucine degradation; valine, leucine and isoleucine biosynthesis; pentose phosphate pathway; tyrosine metabolism; starch and sucrose metabolism; primary bile and biosynthesis; and tryptophan metabolism (Table 4 and Fig. 4B).
DISCUSSION
In lactating dairy cows with NEB, the increased glycine concentration in plasma is related to the breakdown of muscle protein [31] or to the de novo synthesis of glycine from threonine and serine [32]. Shibano et al. [33] reported that glycine in the serum could be used as a marker for EB and metabolic position in lactating dairy cows. In addition, the ratio of glycine to alanine concentration in the serum was used as a biomarker for malnutrition in lactating dairy cows during early lactation [33]. In this study, glycine concentration was higher in the SCK group. Alanine concentration was higher in the CON group; however, not significance different (p > 0.05). High concentration of glycine, kynurenine, and pantothenate and low concentration of arginine as a novel biomarker for NEB diagnosis [34]. In addition, the low concentration of arginine in lactating dairy cows with NEB induces an increase in nitrogen oxide concentration with an attendant increase in blood flow, which is useful for higher nutrients supply for milk production in the mammary gland [35]. In this study, pantothenate concentration was higher in the SCK group; however, not significance different (p > 0.05). Arginine was quantified only CON group and the VIP score was high in the CON group. The substrates for de novo synthesis of FA are BHB and acetate and are used by the mammary epithelial cells to synthesize short and medium chain FAs and sixteen-carbon FAs [36]. During the NEB period, the de novo synthesis of FA decreases and the body commence to use its own storage of energy [37]. In addition, type I and II ketosis have higher concentrations of acetate and BHB [14]. In this study, BHB concentration was higher in the SCK group; however, not significance different (p- > 0.05), and the acetate concentration in the SCK group was tended to be higher (0.05 ≤ p < 0.1) compared with the CON group. Low concentrations of blood glucose are related to hypoinsulinemia, which subsequently activates FA mobilization from tissues, thereby increasing the concentration of ketone body metabolites [7]. Carocho et al. [38] reported that the caloric of 100 g glucose and sucrose led to different peaks in blood glucose concentration. Sucrose is a disaccharide, comprising one molecule of glucose and one molecule of fructose [39]. Therefore, sucrose is connected to the concentration of glucose in the blood. In this study, glucose concentration was higher in the CON group; however, the difference was not significant (p > 0.05). In addition, sucrose was quantified in the CON group but not in the SCK group, and the VIP score was also higher in the CON group. Blood BHB, acetoacetate, and acetone are associated with the incomplete beta-oxidation of mobilized excess of fats that, result in ketosis [40,41]. Among them, BHB and acetone concentrations in the plasma are useful in the diagnosis of SCK in lactating dairy cows during early lactation [42,43]. In this study, BHB and acetone concentrations were higher in the SCK group; however, the difference was not significant (p > 0.05). Acetoacetate concentration was significantly (p- < 0.05; VIP score : 1.85) higher in the SCK group.
5-ALA is a dietary supplement for livestock that can affect the synthesis of heme and positively influence the iron status of hemoglobin in animals [44]. In addition, 5-ALA supplementation improved milk protein, fat and casein in dairy cows [45,46]. 5-ALA in blood is a product of condensing succinyl-CoA and glycine through the catalytic activity of 5-ALA synthase [47]. Hendawy et al. [48] reviewed biological activities of 5-ALA and reported, for example, antioxidant, anti-inflammatory, and immunomodulator activities. Nuclear factor kappa-light-chain-enhancer of activated B cells (NF-κβ) induces a variety of genes that encode proteins involved in inflammation including tumor necrosis factor (TNF), interleukin-1 (IL-1), and interleukin-23 [49]. Betaine supplementation improved milk yield, fat and FA synthesis [50]. Betaine in serum has shown several anti-inflammatory effects including the inhibition of NF-κβ [49]. Ametaj et al. [51] reported a potential role of immune factors in triggering systemic inflammation during the transition period in the pathobiology of metabolic disorders (e.g, concurrent disease with type II ketosis). In this study, 5-ALA (p < 0.01; VIP score : 1.67) and betaine (p < 0.05; VIP score : 1.60) concentration were significantly lower in the SCK group. Therefore, 5-ALA and betaine levels in the serum of lactating dairy cows are potential biomarkers for the diagnosis of ketosis. However, further research is needed on the relationship between the two metabolite (5-ALA and betaine) and ketosis diagnosis.
SCK involves an increase in the levels of ketone body metabolites in the urine, and the distinct signs of CK disease are absent [7]. Recently, the acetoacetate level in urine was used as a biomarker for ketosis diagnosis in the dairy industry [15]. This method is a quantitative test limited by its short sensitivity, and is used only for examine purposes [52]. In this study, acetoacetate and acetone concentrations were higher in the SCK group; however, the difference was not significantly (p > 0.05). The BHB concentration tended to be higher (0.05 ≤ p < 0.1), as did the VIP score (1.54) was also higher in the SCK group. Kawasaki et al. [53] reported that urine fructose concentration decreases during ketosis in patients. Acute administration of fructose promotes other adverse metabolic diseases, including hyperuricemia and lactic acidosis [54]. Therefore, the fructose level in the urine of lactating dairy cows might be considered a potential biomarker for the diagnosis of ketosis. In this study, fructose concentration was higher in the CON group; however, the difference was not significantly (p > 0.05). Amino acids are substantial precursors for associated with gluconeogenesis and ketogenesis [55,56], and crucial moderator or intervening in diverse metabolic pathways, including cell signaling, immunity, growth, maintenance and oxidative stress [57–59]. Therefore, amino acid metabolism is essential for sustained condition and for preventing diseases (metabolic and contagious) [15]. Pantothenate has importance metabolites in production of carbohydrate and FA metabolism associated with energy [60]. Zhang et al. [15] reported that amino acid metabolites (arginine, aspartic acid, glutamate, glycine, alanine, cysteine, isoleucine, lysine, phenylalanine, and tyrosine) and carnosine, N-acetylglutamate, 1-methylhistidine, 3-methylhistidine and pantothenate were higher concentration in the urine of normal cow. In this study, glycine, 3-methylhistidine and pantothenate concentrations were higher in the CON group; however, the difference was not significantly (p > 0.05). Alanine concentration tended to be higher (0.05 ≤ p < 0.1) in the CON group. In addition, aspartate and glutamate metabolites was not significance different (p > 0.05) between the two groups.
Homogentisate in urine is produced through the catabolism of phenylalanine by homogentisate 1,2 dioxygenase (HGD) [61], and HGD affects body weight and sirloin cross-sectional area in cattle [62]. Holtenius and Holtenius [10] reported that CK in lactating dairy cows reduced milk yield and body weight. In this study, homogentisate concentration was significantly (p < 0.01; VIP score : 1.81) higher in the CON group. Theophylline is a xanthine-based metabolite and, an intermediate product in the metabolic process of caffeine and 3-MX. This metabolite can be excreted through the kidneys and has a diuretic effect [63,64] and can cause a variety of side effects in cows, such as acid-base and electrolyte imbalances [65]. In this study, theophylline concentration was significantly (p < 0.01; VIP score : 2.01) higher and 3-MX concentration was tended to be higher (0.05 ≤ p < 0.1; VIP score : 1.71) in the SCK group. The p-cresol concentration in urine may reflect the intake of dietary phenylalanine and tyrosine in non-ruminants and thus may be a proxy of the overall N intake [66]. However, approximately half of the tyrosine content of rumen-administered casein was excreted as p-cresol [67]. Therefore, an excessively high concentration in the urine may have a negative effect on nitrogen metabolism in ruminants. In this study, p-cresol concentration was significantly (p < 0.05) higher in the SCK group. Since the research on urine metabolites related to ketosis in lactating dairy cow is insufficient the relationship between metabolites and ketosis shown in this study will be helpful for minimizing the incidence of the disease. In addition, homogentisate, theophylline, 3-MX and p-cresol levels in the urine of lactating dairy cows are potential biomarkers for the diagnosis of ketosis. However, further research is needed on the relationship between the four metabolite (homogentisate, theophylline, 3-MX and p-cresol) and ketosis diagnosis.
The metabolites profiling of CON and SCK group lactating dairy cows were investigated by 1H-NMR spectroscopy. In the serum, associated with inflammation (5-ALA and betaine) and positive energy balance (arginine) metabolites was high concentration in the CON group, whereas ketone bodies including acetoacetate and acetate were high concentration in the SCK group. In the urine, associated with gluconeogenesis (amino acids; alanine) and body weight (homogentisate) were high concentration in the CON group, whereas ketone bodies including BHB was high concentration in SCK group. In Korea, studies on metabolic profiling by 1H-NMR spectroscopy are inadequate. Therefore, this study will contribute to future ketosis metabolomics studies in Korea by serving as a reference guide.