INTRODUCTION
In the current beef industry, intramuscular (IM) fat contents are critical factors in determining the beef quality and economic value. Increasing days on feed with a grain-based diet is a common and widely used strategy to maximize the marbling of beef without modifying the genetics of animals. This feeding strategy can decrease feed efficiency and increase backfat thickness simultaneously. Since energy costs increase as the proportion of gain as internal or subcutaneous (SC) fat [1], an increased amount of that adipose tissue may be less desirable. There have been efforts to increase IM fat intensively without altering other, economically valueless adipose tissues. With the long-period selection, Wagyu and Hanwoo cattle showed high-marbling traits with less backfat thickness while feeding grain-based diets with around 30-month of the feeding period. At the same time, researchers also have been searching for nutritional methods to increase IM fat, such as a vitamin A-deficient diet. The long-term depletion of vitamin A has commonly been used in a few countries to increase IM adipose tissue accumulation [2]. Multiple vitamin A isoforms, including 9- cis retinoic acid and all-trans-retinoic acid reported as an inhibitor of peroxisome proliferator-activated receptor γ (PPARγ) and retinoid X receptor (RXR). Among various vitamin A isoforms, all-trans retinoic acid (ATRA) is known to be the most active isoform in mammals. It has numerous physiological effects such as vision, embryonic development, reproduction, hematopoiesis, and differentiation of epithelial and mesenchymal cells. It also reported that dietary vitamin A negatively affects the development of adipose tissues in mammalian animals [3–5]. Therefore, ATRA has been chosen as a vitamin A source in the current study. In countries where marbling is the most important factor for consumer’s beef choice, vitamin A or provitamin A-deficient diets have been used for beef cattle to maximize IM fat accumulation [2]. Inhibitory effects of vitamin A isoforms have been tested with various cell and animal types such as swine [6], cattle [7], and rodents [8,9]. Nevertheless, much is unknown about the biological metamorphosis of adipocytes, which have many differences across species and peripheral adipose depots. Therefore, this study was conducted to determine the influence of ATRA on genes associated with adipogenic differentiation of bovine SC and IM adipocytes.
MATERIALS AND METHODS
IM preadipocytes were isolated from three 16- month- old, crossbred steers (predominantly Angus, 474.5 ± 50.2 kg). Cattle were harvested in the Meat Science Laboratory (abattoir) at Texas Tech (Lubbock, TX, USA) under United States Department of Agriculture (USDA) inspection and exsanguinated following captive bolt stunning. Bovine IM adipose tissue was collected from between the 10th and 13th rib sections of the longissimus dorsi (LD) muscle (Fig. 1). SC adipose tissue was collected from the 10th and 13th rib areas immediately after the skin was removed. Isolated LD muscle SC adipose tissue and a chunk were promptly transported to the Texas Tech University Meat Science and Muscle Biology Laboratory (Lubbock, TX) in cold sterilized phosphate-buffered saline (PBS; Gibco, Waltham, MA, USA) with 3 × Antibiotic-Antimycotic (Gibco). The IM fat tissue was dissected from LD, excluding connective tissue, blood vessels, and skin was removed. Both IM and SC Adipose tissues were minced finely and incubated in the warm digestion medium, containing Earl’s Balanced Salt Solution (EBSS, Sigma-Aldrich, St. Louis, MO, USA), 0.1% of type IV collagenase (Thermo Fisher Science, Waltham, MA, USA), and 1 × Antibiotic-Antimycotic (Gibco) for 40 minutes at 38°C. The collagenase-digested adipose tissue was filtered through a 100 μm nylon membrane, and the suspension was then centrifuged for 5 min at 2,000×G. After discarding the supernatant and lipid layer, the pellet was washed 3 times in Dulbecco’s Modified Eagle Medium (DMEM). The pellet was re-suspended and maintained in growth media composed of DMEM (Gibco), 10% fetal bovine serum (FBS, Gibco), and 1× Antibiotic-Antimycotic (Gibco) at 37°C under a humidified atmosphere of 5% CO2. Upon reaching approximately 90% confluency, the growth medium was replaced by differentiation media composed of DMEM (Gibco), 5% FBS (Gibco), 10 μg/mL insulin (Sigma-Aldrich), 10 μg/mL hydrocortisone (Sigma-Aldrich), 5 μM ciglitizone (Sigma-Aldrich), and 1× Antibiotic-Antimycotic (Gibco) with doses of 0 (Con), 0.01, 0.1, 0.1, 1 μM, and 10 μM of ATRA (Sigma-Aldrich). In addition to this, 1 μM of retinoic acid receptor (RAR) antagonist (ANT; AGN 193109 sodium salt, Santa Cruz Biotechnology, Dallas, TX, USA) was used in a dose-dependent manner, 0 (Con), 0.01, 0.1, 1 μM, and 10 μM for 24 h and 96 h of incubation.
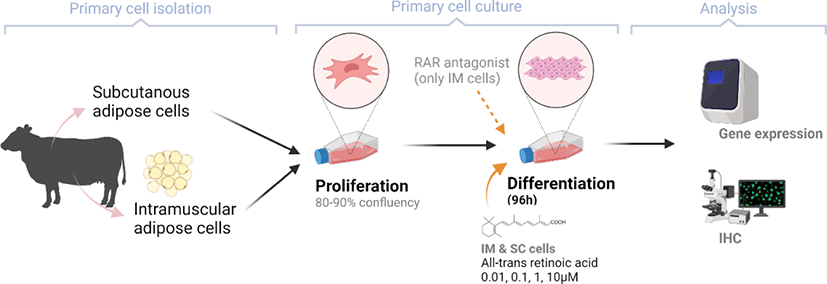
After 96 h of differentiation, the plates were removed from the incubator and rinsed three times with PBS. The fixation of IM cells was conducted using 10% formalin (Sigma-Aldrich) for 10 min. Lipid droplets were stained using a 0.5% Oil Red O solution (ORO, Sigma-Aldrich) in propylene glycol (Sigma). After 1h of incubation with ORO solution in a dark room, plates were rinsed with de-ionized (DI) water. Plates were then stained using Mayer’s hematoxylin (Sigma-Aldrich) for 20 min to visualize nuclei. All cells were imaged with a Nikon Eclipse Ti-U microscope (Nikon Instruments, Tokyo, Japan). Images were processed using NIS-Elements software (Nikon Instruments).
Cultured IM and SC preadipocytes were trypsinized (Sigma-Aldrich) and centrifuged for 5 min at 300×g. Total RNA isolation and purification steps were performed using the RNeasy® Mini kit (Qiagen, Germantown, MD, USA) according to manufacturer recommendation. The concentration and purity of RNA were measured with a spectrophotometer at an absorbance of 260 nm and 280 nm using a NanoDrop 1000 (NanoDrop Technologies, Wilmington, DE, USA). An acceptable range of 1.76 to 2.05 was used for the 260/280 ratio. Genomic DNA removal and cDNA synthesis were conducted using QuantiTect reverse transcription kit (Qiagen) according to manufacturer recommendations. Real-time quantitative PCR (7900-HT Real-Time PCR System, Applied Biosystems, Foster City, CA, USA) was used to measure the quantity of CCAAT/Enhancer binding protein (C/EBP) β, PPARγ, glucose transporter 4 (GLUT4), stearoyl CoA desaturase (SCD), and Smad transcription factor 3 (SMAD3) relative to the quantity of ribosomal protein subunit 9 (RPS 9) mRNA in total RNA (Table 1). Since the expression of RPS9 did not differ across samples nor treatments, RPS9 was used as the endogenous control in order to normalize the expression of genes. Measurement of the relative quantity of the cDNA of interest was carried out using TAMRA PCR Master Mix (Applied Biosystems) using the appropriate forward and reverse primers and cDNA mixture. Assays were performed in triplicate determinations using the thermal cycling parameters recommended by the manufacturer (40 cycles of 15 s at 95°C and 1 min at 60°C). Titration of mRNA primers against increasing amounts of cDNA yielded linear responses with slopes between −2.8 and −3.0. The RQ manager (Applied Biosystems) analyzed real-time quantitative (RQ) values based on ΔΔCT.
Cells were grown on 3-well microscopy glass slides (Ibidi USA, Fitchburg, WI, USA) for 96 h after inducing differentiation. Slides were fixed with cold 4 % paraformaldehyde (Thermo Fisher Scientific) for 10 min at room temperature. In order to prevent nonspecific background staining, fixed cells were incubated in the blocking solution containing 2% bovine serum albumin (Thermo Fisher Scientific), 5% of horse serum (Thermo Fisher Scientific), and 0.2% Triton X-100 in PBS for 30 min at room temperature. Fixed cells were then incubated with primary antibody anti-PPARγ (rabbit polyclonal, dilution 1:100; Abcam, Cambridge, UK) at 4°C overnight.
The colorization of F-actin was conducted using Bodipy (558/568) phalloidin (Thermo Fisher Scientific) according to the manufacture’s guidance. Nuclei were stained with 4’,6-diamidino-2-phenylindole (DAPI, Thermo Fisher Scientific) for 5 min. Slides were imaged at a magnification of 20X using an inverted fluorescence microscope (Nikon Eclipse, Ti-E; Nikon Instruments) equipped with a UV light source (C-HGFIE, Nikon Intensilight, Tokyo, Japan). The NIS Elements® imaging software analyzed all images.
Gene expression data were analyzed as a completely randomized design using the MIXED procedure of SAS 9.4 (SAS Inst., Cary, NC, USA) using orthogonal contrasts for the dose-effect analysis. All results were reported as least-squares means. An α level of 0.05 was used to determine significance, with tendencies discussed at P-values between 0.05 and 0.10.
RESULTS AND DISCUSSION
IM adipose tissue, also known as marbling fat in the meat industry, displays distinctive characteristics from other adipose tissues, primarily through their unique location and cellular level metabolism [4]. IM adipose cells are located within the perimysium alongside myofibers [10]. Due to its unique location, it is considered that this adipose tissue may closely communicate with neighboring muscle cells.
There are two distinctive phases during adipogenesis, which are determination (proliferation) and differentiation. During the determination period, adipocyte precursor cells and preadipocytes stay in the cell cycle and increase numbers through mitosis. While the differentiation process of preadipocytes into mature adipocytes is well-studied, a large portion of adipogenic determination is still unknown. Various transcriptional regulation events occur during adipogenic differentiation. The majority of them are associated mainly with the PPARγ or C/EBP family, which are often referred to as key transcription factors [4,11]. Among various C/EBP isoforms, C/EBPβ plays a crucial role in the early stage of adipogenesis. In our current study, a high dose of ATRA (10µM) suppressed (p < 0.05) the expression of C/EBPβ in SC cells (Fig. 5). Unlike the SC cells, ATRA treatment did not alter (p > 0.05) the C/EBPβ gene expression in IM (Fig. 4). The expression of C/EBPβ is almost simultaneous with the induction of adipogenic stimuli. C/EBPβ induction leads to PPARγ activation, the master regulator of adipogenic differentiation, binding to their promoters [12]. In SC cells, the PPARγ level tended to decrease (p < 0.1) in 1 and 10µM compared to Cont. In IM cells, the addition of 10µM of ATRA down-regulated (p < 0.05) expression of PPARγ compared to Cont. The difference in C/EBPβ expression may be one of the distinguishing characteristics of IM adipocytes. The expression of C/EBPβ in preadipocytes is initially low but dramatically increases during the early stage of terminal differentiation [13]. PPAR, a member of the nuclear hormone receptor superfamily, play a significant role in various types of cell metabolism. Three different PPAR isoforms, PPARγ, PPARa, and PPARd have been identified. Each of these isoforms is involved in diverse physiological phenomena [14]. PPARγ plays an essential role during adipogenic differentiation and simultaneously controls lipid metabolism-related gene expression. In lipid and glucose metabolism, synthetic PPARγ ligands, including troglitazone, rosiglitazone, and pioglitazone, stimulate glucose uptake and improve insulin sensitivity in adipocytes, hepatocytes, and skeletal muscle cells [11,15]. Adipogenesis is stimulated upon the activation of PPARγ via ligands, and the mRNA level of PPARγ increases during bovine SC preadipocyte differentiation [16,17]. The inhibitory action of ATRA on adipogenic differentiation seems to be closely related to PPARγ. To regulate the terminal differentiation of adipose cells, PPARγ must heterodimerize with another nuclear hormone receptor, RXR [18]. Only ATRA activates RAR moiety by binding to the ATRA response element, but 9-cis-retinoic acid can bind RXR and RAR [3]. Once ATRA binds to RAR with a high affinity and mediates the RAR/RXR heterodimer, PPARγ is unable to form the PPAR/RXR heterodimer. Several researchers have verified this series of PPARγ – involved adipogenesis blocking processes in recent decades [3,7,8]. To demonstrate the role of ATRA as an antagonist of RXR-PPARγ heterodimer formation, we tested a RAR antagonist. The results showed that the RAR antagonist activated (p < 0.05) PPARγ genes with ATRA presence while C/EBPβ. RAR antagonist seemed to accelerate the adipogenic differentiation when the high dose was applied. At the point that cells were harvested, the expression of PPARγ was mediated by increased C/EBPβ and C/EBPβ is started to be downregulated in 10 µM of RAR antagonist treated cells. The expression of C/EBPβ is rapidly increased in the early stage of differentiation, and the peak of expression of C/EBPβ preceded the accumulation of PPARγ mRNA and then diminished subsequently [11]. This is also confirmed by morphological analysis. RAR antagonist treatment group showed lipid droplet accumulation (Figs. 2 and 3). These sequential events transcriptionally activate genes associated with the adipogenic phenotype [19,20].
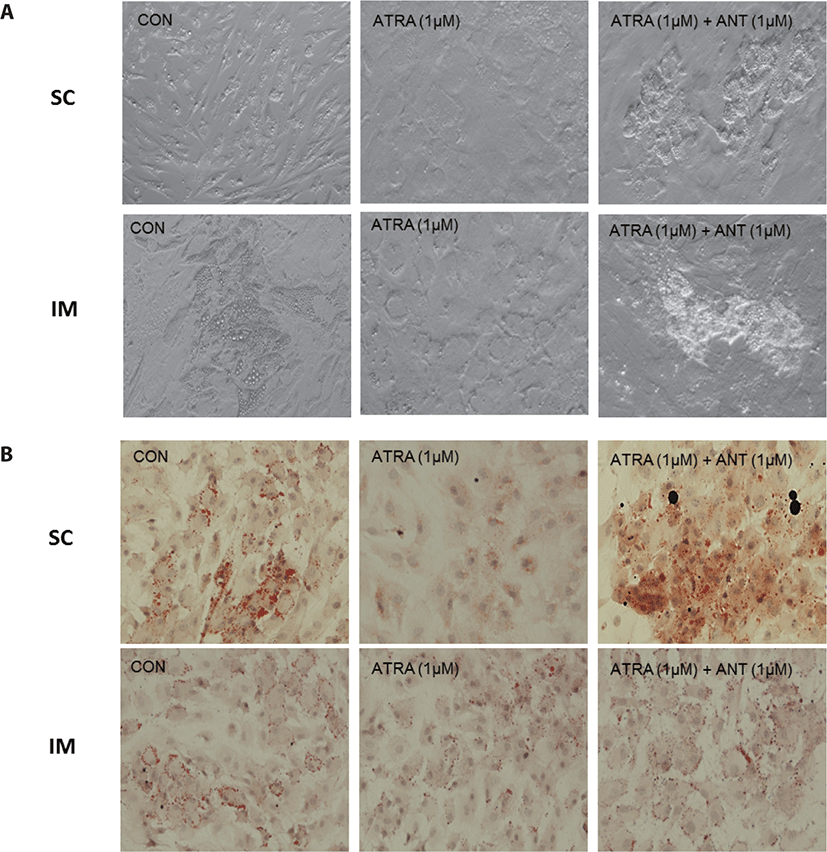
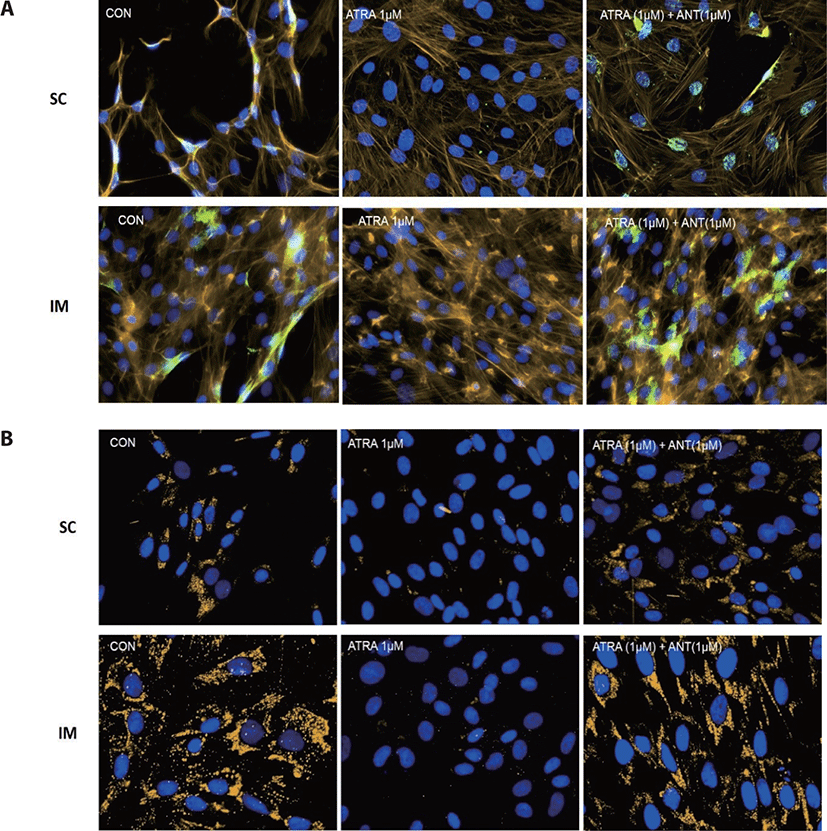
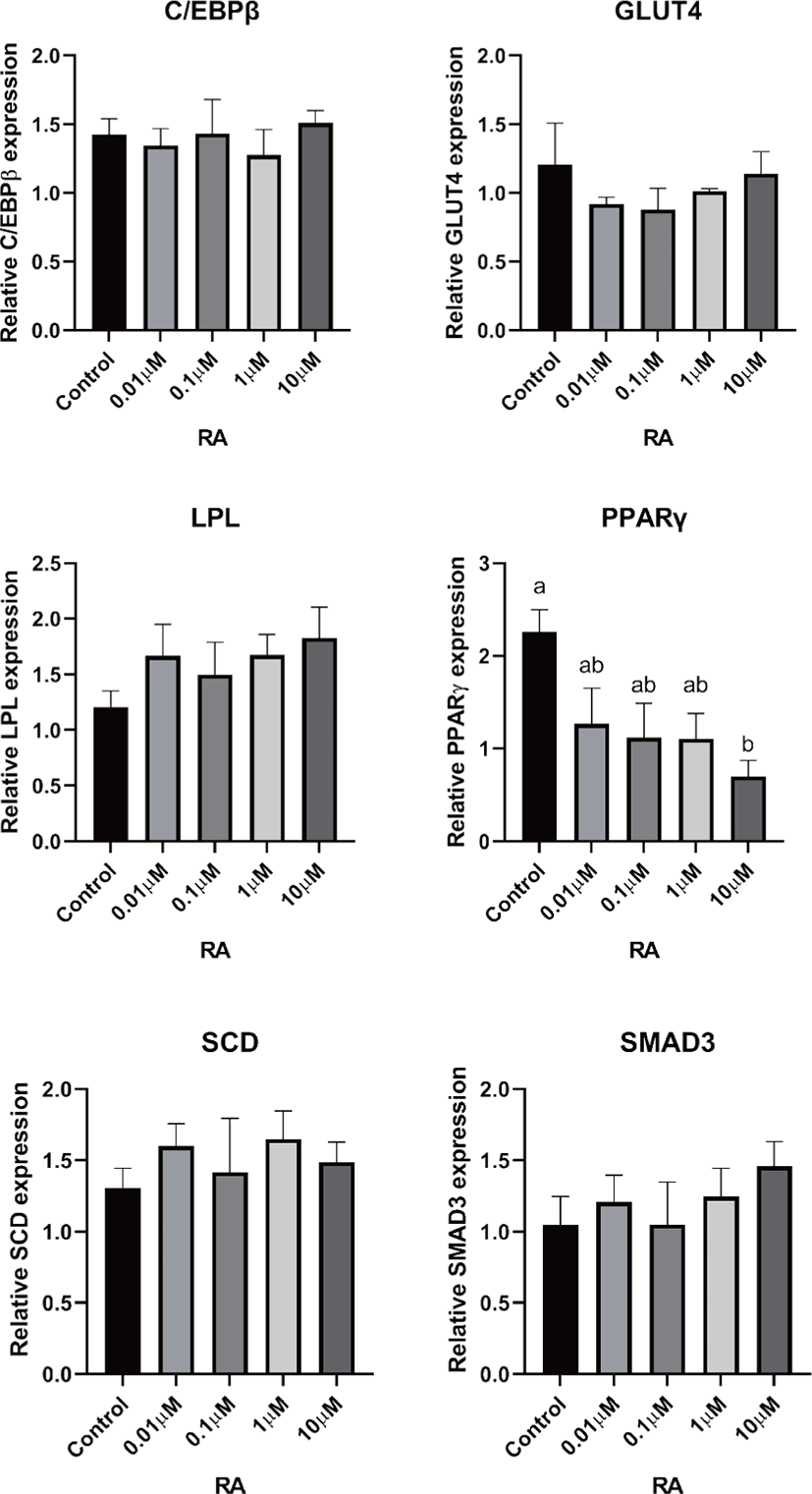
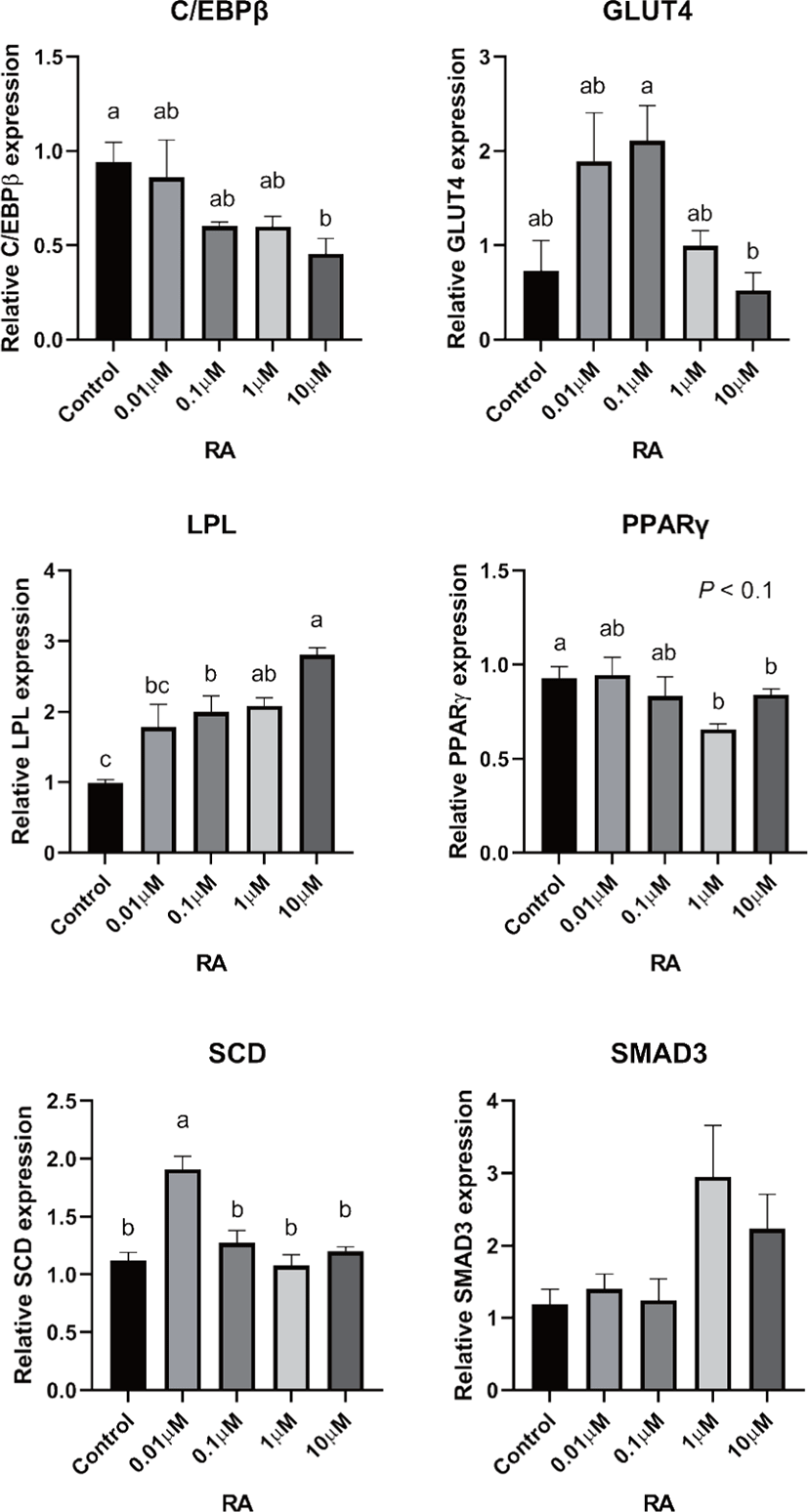
In the previous swine study, PPARγ expression was lower in IM adipocytes than SC or peripheral adipocytes and other genes associated with lipogenesis, including fatty acid synthase and malic enzyme [21]. It seems evident that IM and SC possess noticeable characteristics in a lipid metabolic and growth manner. First, IM and SC appear to utilize different types of substrates for lipogenesis. Smith and Crouse [22] suggested that carbon sources for the fatty acid biosynthesis differed across locations in the body; IM adipocytes preferentially used glucose, while SC adipocytes utilized acetate as a fuel source. In addition to this, IM adipose tissue used palmitic acid for triglyceride synthesis, distinctly different from SC adipose tissue [23]. Second, IM adipocytes generally show less metabolic activities compared to adipocytes in other locations such as SC and perirenal throughout the animal’s growth [4]. Understanding the physiological characteristics of adipose cells in different locations, especially IM and SC, is crucial because this fat depot is directly related to the economic value of beef in many grading systems [24]. Further studies are required to develop the feeding strategy to increase IM fat without affecting the SC fat.
The addition of ATRA also tended to modify (p < 0.01) SMAD3 gene expression in both IM and SC adipocytes. The role of SMAD3 in the suppression of adipogenic differentiation by ATRA seems critical. It regulates adipogenesis by altering the expression of C/EBPβ. Marchildon, St-Louis [25] indicated that ATRA does not directly regulate C/EBPβ but indirectly stimulates the expression of the Smad3. They also identified that in the absence of Smad3, ATRA was not able to inhibit adipocyte differentiation or elicit a decrease in C/EBP. A disturbance in C/EBPβ binding to DNA response elements in promoters can occur due to the ability of Smad3 to bind to this bzip transcription factor [26]. Hence, Smad3 could be another substance involved in the inhibition of adipogenesis via ATRA. The ATRA treatment-induced Smad3 protein expression in 3T3-L1 cells regardless of the co-treatment with the adipogenic mixture used to induce C/EBPβ expression and differentiation [8].
In previous studies, retinoic acid treatments increased plasma triglycerides in human and rodent models [27,28]. Previous studies demonstrated that retinoic acid did not directly change LPL mRNA gene expression in 3T3-F442A [29] and primary adipose cells isolated from epididymal fat of rats [27]. ATRA may alter LPL activity in adipose tissue; however, it may be due to the secondary effect of retinoic acid but the direct regulation of the LPL gene [27]. In agreement with the previous study, our data from SC cells also indicated that the addition of ATRA did not alter LPL gene expression. However, the LPL mRNA gene expression was increased in 10 µM of LPL compared to 0, 0.01, and 0.1 µM of ATRA treatment (Fig. 5). Blaner and coworkers [30] reported that retinyl esters are can be substrates for LPL and suggested that LPL involve in delivering retinol to adipocytes. However, data related direct effects of ATRA on LPL gene expression on IM cells is lacking. Additional studies are required.
The ATRA treatment did not alter (p > 0.05) the expression of SCD in IM cells, but 0.01 µM of ATRA increased (p < 0.05) SCD in SC cells. The expression of the SCD gene is considered a later marker in adipocyte differentiation [26]. Enzyme activity and SCD gene expression in bovine adipose tissues were reported as an indicator of fat softness and were an essential aspect of meat quality [31,32]. Kruk et al. [33] reported that dietary vitamin A restriction elevated SCD activity and enhanced marbling scores simultaneously in beef cattle. These data provide additional evidence that SCD activity is highly correlated with marbling scores. The level of SCD gene expression and SCD enzyme activity is closely associated with adipocyte differentiation in bovine IM adipose tissue [34]. In the current study, treatment of ATRA on IM did not alter the expression of SCD. Because SCD is considered a late-expression gene among adipogenic markers, 96 h of incubation in differentiation media may not be long enough to detect changes in SCD gene expression in IM. Contrary to this, ATRA increased SCD expression in SC adipocytes. A low concentration (0.01 µM), possibly lower than average blood retinoic acid concentration, may increase SCD expression in SC adipocytes.
The transporter associated with glucose uptake in adipose tissue, GLUT4, gene expression was not affected by ATRA treatment in IM adipocytes. However, 0.1 µM of ATRA upregulated (p < 0.05) the expression of GLUT4 in SC cells. A similar tendency was observed in the studies with the muscle cell. The expression of GLUT4 mRNA level was upregulated when RA was treated [35,36].
Glucose transporter 4 (GLUT4) is the major glucose translocator from the plasma membrane to intracellular storage that mainly expresses insulin-targeted tissues such as adipose and muscle tissue. There was no significant difference (p > 0.05) in gene expression of GLUT4 in IM adipocytes (Fig. 6). The expression GLUT4 was the greatest (p < 0.05) in the SC cells when 0.1µM of ATRA was added (Fig. 5). Direct effects of ATRA on GLUT4 activity in adipose cells seemed not clear. There are a few suggested indirect pathways that ATRA or other vitamin A isoforms have an impact on glucose transportation in adipose cells. Janke et al. [37] reported that retinol-binding protein 4 (RBP4), the transporter of retinol, has a positive correlation with GLUT4 in human adipose cells. Another suggested glucose uptake regulation of ATRA was via RAR signaling in the pancreas and mediated the insulin secretion [38].
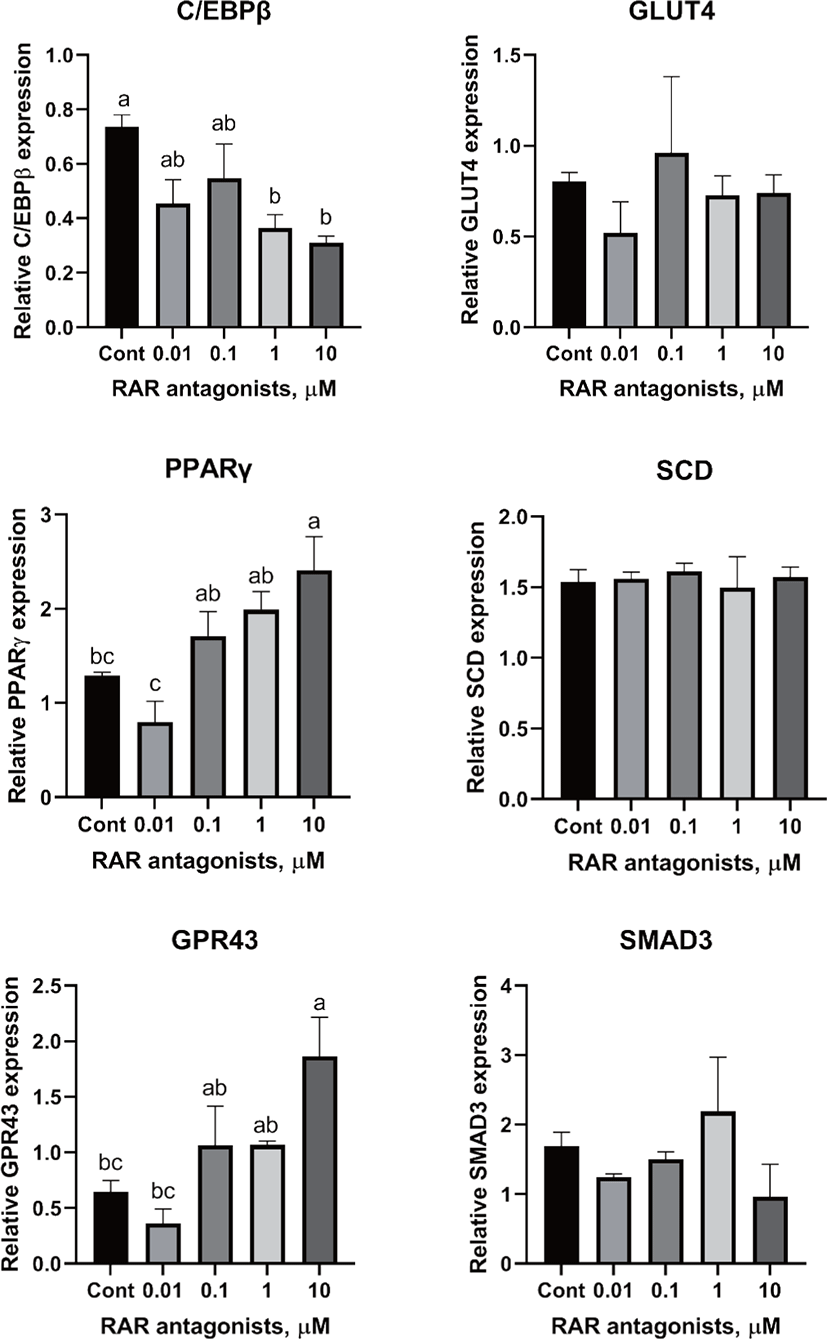
CONCLUSION
Vitamin A deficient diet has long been used to improve IM fat contents in beef cattle in some countries. Our current study demonstrated that adipogenic differentiation could be blocked by downregulation of the genes such as C/EBPβ (SC cells) and PPARγ (IM- tendency, SC) when high doses of ATRA were added.
In some feeding systems, vitamin A is restricted during the fattening stage. The standard fattening period for beef cattle lasts more than one-third of the lifespan; therefore, vitamin A consumption may be restricted for an extended period. Offering a minimum amount of provitamin or carotene is required to prevent health issues caused by vitamin A deficiency.