INTRODUCTION
In vitro cultured meat production is an innovative concept based on tissue engineering and myogenesis without slaughtering of livestock [1–3]. These meats are produced from muscle satellite (stem) cells (MSCs), which are being widely used for this purpose because they proliferate and differentiate efficiently and are primary generators of new myonuclei [4]. MSCs are important for the maintenance of skeletal muscle (SM) structure and function, and meat is largely composed of SM. The SM contains 90% muscle fibers and 10% connective and fat tissues [5–8]. Furthermore, meat produced using MSCs represents an excellent new source of high-quality protein [9–11]. MSC self-renewal capacity is important for maintaining MSC populations and generating enormous numbers of myogenic cells, which proliferate, divide, fuse, and contribute to the synthesis of new myofibers [12]. Cultured meat was initially produced using bovine MSCs [13], and considerable research has been undertaken in recent years to produce functional meat products [14].
Myogenesis is a term used for the process leading from the development to the formation of SM tissue and involves MSC activation and proliferation (due to the expressions of muscle-specific genes and cell cycle-related factors) and the fusion of differentiating myoblasts into mature myofibers (under the direction of muscle regulatory factors). Myogenesis is mediated by MSCs and is responsible for the normal growth and repair of SM. This process is regulated by myogenic regulatory factors such as Pax3, Pax7, Myf5, MyoD, MyoG, and Myf6 [15,16] (Fig. 1).
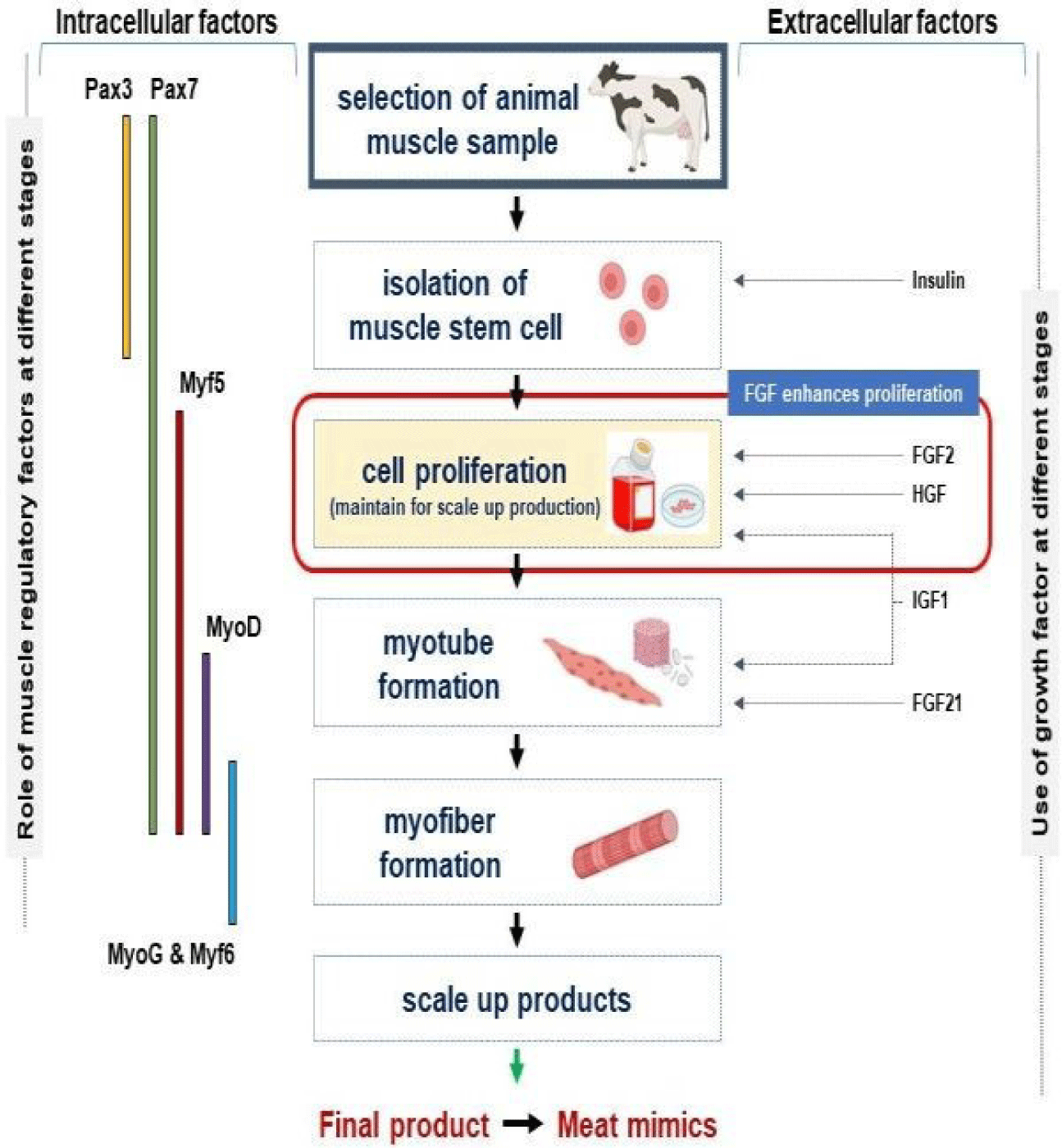
Furthermore, MSC culture [17,18] and the directed differentiation of pluripotent MSC [19,20] provide novel approaches to in vitro myogenesis. Several extracellular matrix (ECM) proteins such as fibromodulin (FMOD) [7,21], matrix gla protein [22], and dermatopontin (DPT) [23] have been reported to regulate myogenesis, and ECM contains molecules such as collagen, integrin, decorin, biglycan, DPT, FMOD, fibronectin, glycosaminoglycan, laminin, and dystrophin that provide structural support, cellular communication, and contribute much to the architectural maintenance of SM [5,24].
Cultured meat is an attractive option compared with regular meat as it is free from environmental pollution, ethical issues, and food security challenges [25–27]. However, several issues, such as color, texture, flavor, and nutritional value, which all depend on protein and intramuscular fat contents, remain to be resolved [28,29]. Cattle, chickens, pigs, and fish have been reported to be suitable target species for biopsy leading to cultured meat production [25], and it has been established that a single biopsy may replace the killing of 20 animals [30].
The different types of MSC, namely, adult, pluripotent (PSCs; embryonic MSC), and induced pluripotent MSC) are used to produce cultured meat [31]. MSCs and adipocytes are required for initiating cultured meat production and, in combination, may contribute to achieving an original meat flavor and texture [12,32,33]. Porcine SM multipotent progenitor cells have a greater doubling capacity than MSCs, but they require expensive recombinant growth factors (GFs) and do not develop into SM fibers as effectively as MSCs [34]. Accordingly, MSCs are the most common source used for cultured meat production, and PSCs (embryonic stem cell [ESC] and iPSC) are considered a second option.
Technical difficulties related to culture media, such as GF, hormones, identification of animals (age and health), sampling of MSCs, consumer acceptance, and religious standpoints, pose considerable challenges to the establishment of viable large-scale in vitro meat production systems, and thus, more research is required before this meat culturing system can be implemented at industrial levels.
MSC proliferation and differentiation are influenced by many GFs such as fibroblast growth factor (FGF), insulin-like growth factors (IGF-1 and 2), transforming growth factor-beta (TGF-β) [35], platelet-derived growth factor (PDGF), and hepatocyte growth factor (HGF). GFs can manage growth signal responses during development and are highly active during cell proliferation and differentiation [16,36–38]. The roles of several GFs and hormones during myogenesis for cultured meat production are summarized in Table 1. Three key hormone types related to the physiological system, that is, testosterone (promotes cellular growth and repair), growth hormone, and glucocorticoids, participate in cultured meat production [39]. The roles of GFs and expressions of muscle regulatory factors at different myogenesis stages are shown in Fig. 1, and a schematic of the process from MSC isolation to muscle fiber development is provided in Fig. 1.
Supplements | Function | Embryonic MSC | Myogenic progenitor cells | Tissue engineering | References |
---|---|---|---|---|---|
IGF-1 | ▪ Induces the proliferation and differentiation of cultured myoblast and promotes tissue growth and development | V | V | V | [40–44] |
IGF-2 | ▪ Stimulate both proliferation and differentiation of myoblasts and MSC. | V | V | [42,45] | |
TGF-β | ▪ Helps in collagen type I synthesis in the ECM, and supporting myofiber development | V | V | [46–48] | |
FGF2 |
▪ Activate myogenic progenitor cells and promotes proliferation and growth of mouse myoblasts. ▪ Upregulates Myf5 and MyoD. ▪ Increases the MSC proliferation. |
V | V | V | [49–52] |
FGF21 | ▪ Involved in cell proliferation and differentiation. | V | [53,54] | ||
Insulin |
▪ Promotes MSC survival, self-renewal, and myoblast growth. ▪ Regulators of muscle protein synthesis and hypertrophy. |
V | V | [43,55–58] | |
HGF | ▪ Activation of quiescent MSC in vivo upon muscle injury. | V | V | [52,59,60] | |
Testosterone | ▪ Increase in muscle fiber size and MSC number. | [61–63] | |||
Transferrin | ▪ Stimulate myogenesis and terminal differentiation in fast chicken muscle during embryonic development. | V | V | V | [64,65] |
Heme protein (Hemoglobin and myoglobin) |
▪ Provides typical color of meat and slightly metallic taste of beef. ▪ Increase the iron content in the product. |
[66,67] | |||
BMP | ▪ Preserve the progenitor pool by inhibiting the Myf5 and MyoD expression. | [68] | |||
mTOR | ▪ Regulate MSC activity and myogenesis by upregulating the expression of Pax7, Myf5, MyoD, and myogenin. | [69,70] | |||
Creatine | ▪ Reduces muscle damage by decreasing the inflammatory response and oxidative stress, and activating MSC. Helps in myopathies. | [71,72] | |||
PDGF | ▪ Increases and improve MSC proliferation. | V | V | [68,73,74] | |
Thyroxine | ▪ Helps to myotube in maintaining their differentiated state for a longer period. | V | V | [57] |
GF, growth factor; IGF, insulin-like growth factors; TGF, transforming growth factor; FGF, fibroblast growth factor; PDGF, platelet-derived growth factors; HGF, hepatocyte growth factor; BMP, bone morphogenetic protein; mTOR, mechanistic target of rapamycin; MSC, muscle satellite (stem) cell; ECM, extracellular matrix.
Types of GFs and hormones in culture medium critically maintain cell proliferation and differentiation, and it has been demonstrated that in their absence, in vitro cultured MSCs lose their stemness [4]. Thus, understanding the properties of MSCs and the effects of GFs and hormones that control myogenesis is critical for the mass production of cultured meat. Previous studies have shown that GFs and hormones are involved in myogenesis, and thus, in this review, we focus on how GFs and hormones exert their influences throughout the stages of myogenesis. The objective of this article was to summarize the roles of different GFs and hormones during MSC proliferation and differentiation for in vitro cultured meat production.
ROLES OF GROWTH FACTORS IN MYOGENESIS FOR CULTURED MEAT PRODUCTION
FGFs are peptide hormones that belong to a large family of polypeptides locally present in developing tissues [75] and are secreted by liver, adipocytes, pancreas, and SM [76]. The FGF family is composed of 22 ligands that interact with four different FGF receptors, and FGF/FGFR signaling governs cell survival, proliferation, migration, differentiation, and tissue repair/regeneration [77]. FGF-21 is actively involved in cell proliferation and differentiation [53]. On the other hand, FGF-2 (basic FGF) is secreted by fibroblasts and has been used extensively for in vitro MSC culture. FGF-2 supplementation increased mouse myoblast growth by 25%, but the removal of FGF-2 from culture media increased the differentiation of myoblast cells. Also, the endogenous secretion of FGF-1 (acidic FGF) by MSCs may trigger cell proliferation and muscle regeneration [78,79]. On the other hand, FGF enhances cell proliferation and differentiation, which play important roles during the initial stage of myogenesis. In addition, FGF-2 also promotes tissue formation and repair by interacting with heparin sulfate cofactor [80].
PDGFs increase the production of three SM basement membrane components, namely, laminin (70%), fibronectin (30%), and type IV collagen (70%). PDGF has four known isoforms (PDGF A, B, C, and D), which are stabilized by intermolecular disulfide bonds. All four PDGF isoforms contain a highly conserved 100 amino acid GF domain [81]. PDGFs are powerful mitogens and affect a wide range of cells, including fibroblasts, smooth muscle cells, connective tissue, bone, and cartilage cells, and have been demonstrated to boost mouse myoblast proliferation. Thus, PDGFs are important components during the production of cell-cultured meat [82].
IGFs play important roles in myogenesis and muscle regeneration by activating MSC proliferation, increasing protein synthesis, and promoting differentiation [83-86]. There are two IGF types, that is, IGF-1 and IGF-2. IGF-1 is a 70 aa long polypeptide that shares ~60% similarity with IGF-2 [87]. IGF-1 was reported to be actively involved in the proliferation and differentiation of myoblast cells [83,88]. The negative effect of myostatin (MSTN) was found to be compensated by the positive effect of IGF-1 during myogenesis [85]. IGF-1 promotes tissue growth and development, stimulates cell proliferation, and has anti-aging, anti-inflammatory, and antioxidant properties [41]. IGF-1 also has direct and indirect glucose-lowering effects, enhances free fatty acid oxidation in muscles, reduces the accumulation of free fatty acid in liver, and insulin signaling, reduces hepatic glucose output, and improves insulin sensitivity [41].
IGF-1 and 2 were reported to be equally effective at promoting chick embryonic myoblast differentiation and fusion [42], whereas IGF-2 was more effective than IGF-1 during turkey embryonic myoblast proliferation and differentiation. IGFs were found to stimulate in chicken and turkey MSC proliferation [89, 90], and treatment with IGF-1 and IGF-2 enhanced MSC growth.
When the effect of IGF-1 on chicken myoblast proliferation was examined IGF-1 dose-dependently increased myoblast numbers. Supplementation with 10, 100, or 1,000 ng/mL of IGF-1 for 24 h increased numbers by 9.5%, 63.1%, and 66%, respectively. The optimal concentration of IGF-1 for stimulating proliferation was found to be 100 ng/mL [40].
Myostatin (growth and development factor-8, MSTN) is present in SM, and muscle mass in MSTN-null mice was reported to be dramatically enhanced due to increased muscle fiber levels (hyperplasia) and sizes (hypertrophy) [91,92]. Furthermore, the double-muscled phenotype in cattle has been linked to the MSTN gene. Muscular hypertrophy in Belgian Blue double-muscled (BBDM) cattle is caused by an 11-base-pare deletion, and this naturally occurring mutation inactivates the MSTN gene and results in a huge increase in SM mass [93]. Cell culture experiments have also revealed that MSTN inhibits the proliferation and differentiation of cultured muscle cell lines and primary myogenic cells [93], which suggests siRNA1 and siRNA5 might reduce MSTN gene expression and boost sheep meat yield [94]. Some natural compounds [95–97] and inhibitory peptides targeting MSTN [21,98], such as Ac-MIF1 and Ac-MIF2-NH2, have been reported to inhibit the effect of MSTN significantly and enhance cell proliferation [99]. Additionally, in vivo and in vitro data showed that FMOD prevents muscle aging by decreasing the activity of MSTN protein [92]. These findings indicate that MSTN gene sequence modification and MSTN inhibition are good strategies for the mass production of cultured meat from agricultural animals.
TGF-β1 is involved in muscle repair through MSC activation, connective tissue development, and immune response regulation [100]. TGF-β1 also attenuated MSC activation when used with HGF and when administered during early MSC activation (0–48 h) or the proliferative phase (48–96 h) TGF-β1 maintained or induced MSC quiescence, respectively, based on MyoD protein levels [101]. TGF-β1 can influence MSC proliferation and myogenesis, enhance mature SM mass, and regulate intramuscular fibrogenesis; it also plays critical roles in muscle formation. TGF-β1 was also demonstrated to restrict myogenesis in 2D cultures while increasing myogenesis in 3D cultures, which makes it a useful regulator of in vitro muscle tissue formation [102,103].
ROLE OF HORMONES IN MYOGENESIS FOR CULTURED MEAT PRODUCTION
Insulin is a hormone that facilitates MSC survival and self-renewal in vitro [55]. Insulin maintains the differentiation power of myoblasts in vitro[104], and in combination with IGF-2, insulin augments the self-renewal property of MSCs [105], which is necessary for the initiation of culture meat production. Insulin works by activating its receptor and the PI3K/AKT cascade to promote cell survival and also activates integrin, which is required for cell survival during niche reformation after passage. In addition, insulin is needed for human ESC survival on E-cadherin-coated surfaces and in suspension, which suggests its involvement in cell-to-cell adhesion [55]. Insulin is a potent mitogen that promotes DNA synthesis and MSC proliferation and has a dose-dependent stimulatory impact on MSC differentiation and fusion. Insulin appears to promote myogenesis and differentiation by boosting the production of myogenin and myosin heavy chain (MHC) isoforms, and insulin administration thickens myotubes, which suggests a role in insulin-induced hypertrophy [106]. Accordingly, insulin is an important factor for cell survival and growth during the initial stage of cultured meat.
Testosterone primarily interacts with androgen receptors in SM to promote muscle growth. In SM, testosterone has a variety of ergogenic, anabolic, and anti-catabolic functions that dose-dependently contribute to muscle strength and hypertrophy [107,108]. In muscle, testosterone promotes muscle hypertrophy by stimulating protein synthesis and inhibiting protein degradation, and when these effects merge, testosterone stimulates muscle hypertrophy. Furthermore, the interaction between testosterone and intracellular androgen receptor modulates specific physiological signals [39,108]. Testosterone dose-dependently increases MSC numbers and muscle fiber sizes [61,62]. A significant increase in myoblasts numbers, which generated bigger myofibers, was observed when MSCs were treated with testosterone. However, the effect of testosterone on myogenesis in chickens and the mechanism by which testosterone regulates SM development remain unclear. In addition, testosterone significantly increased the cross-sectional area and density of myofibers in muscle [62].
Androgens and estrogens also significantly influence muscle physiology and metabolism and are involved in muscle mass development, maintenance, and repair [109]. Although estrogens and androgens both have favorable effects on muscle, androgens play a major role in the control of muscle physiology and also influence muscle mass by decreasing protein breakdown and autophagy [110]. Overall, estrogens and androgens regulate muscle formation and maintenance.
Glucocorticoids, primarily cortisol, have substantial effects on human SM. Cortisol regulates SM energy homeostasis and metabolism [111,112]. Glucocorticoid receptors are present in the ECM of mammalian SM fibers and signal quickly through the mitogen-activated protein kinase pathway [113,114]. Dexamethasone is a synthetic glucocorticoid known for its anti-inflammatory and immunosuppressive properties and is used to suppress muscle degeneration and stabilize muscle strength [115]. At a concentration of 25 nM dexamethasone increased myogenic proliferation [116], and treatment with dexamethasone has also been reported to increase MSC myogenic differentiation marked by advanced sarcomere formation [105,116,117]. Also, the addition of dexamethasone to muscle cell cultures enhances myogenesis.
SM is a primary target of thyroid hormones [118], which are important for stimulating MSC proliferation and differentiation and muscle recovery and myogenesis [119,120]. Thyroid hormones enhance the numbers and diameters of muscle fibers and also help determine muscle fiber distribution patterns [121], but high or low thyroid hormone levels promote muscle atrophy and impede muscle regeneration [121,122]. Peroxisome proliferator-activated receptor gamma coactivator 1-alpha (PPARGC-1α) is a major regulator of mitochondrial production and is significantly up-regulated by thyroid hormones. One study reported that the presence of thyroid hormones enhanced mitochondrial numbers, protein synthesis, and basal O2 consumption, adenosine triphosphate (ATP) turnover, and maximum respiratory capacity [118]. SM contains type 2 and 3 iodothyronine deiodinases (DIO2 and DIO3, respectively). DIO2 is a strictly controlled enzyme that catalyzes the outer-ring monodeiodination of the secreted prohormone tetraiodothyronine (T4) to produce active hormone tri-iodothyronine (T3), which can either stay in myocytes, signal through nuclear receptors, or exit cells and interact with the extracellular pool [121, 123]. Thyroid hormone signaling plays an important role in SM development and regeneration, and T3 signaling is crucial for SM development [123].
ANIMAL TISSUE CULTURE TECHNIQUES
Cell culture is a process whereby cells are grown in engineered environments, and animal cell culture is one of the most widely utilized research techniques in the biological sciences. Using tissue engineering techniques, in vitro meat production provides a unique means of producing meat without using animals [124]. The basic technology for in vitro meat production involves cultivating muscle tissue in a bioreactor. Starter cells for meat production can be obtained by live animal biopsy and then cultured in vitro [124,125]. The development of a basic culture medium has allowed the growth and differentiation of various cells studied under various conditions [126]. Interestingly, optimal in vitro culture temperature, pH, CO2, O2, osmolality, and nutrition requirements may not be identical to in vivo conditions. Furthermore, sterile cultivation conditions, a constant supply of nutrients, and complex incubation conditions are required [127]. Although nutrients, adhesion proteins, and GFs are abundant in fetal bovine serum (FBS), the exploration of alternative media and the creation of serum-free media formulations have attracted global interest due to shortcomings in in vitro data quality and repeatability and animal welfare issues. The majority of studies on the topic indicate that chemically specific serum-free mediums are the ultimate goal [128, 129]. Research efforts continue to be directed toward the development of optimal culture conditions that improve the efficacy of cultured meat production.
GFs are signaling molecules that stimulate growth, proliferation, and differentiation by triggering signaling cascades after binding to their receptors. GFs are important cell culture media components and provide signals for growth, structural stability, and cell-to-cell communication. However, GFs are expensive, and thus, some alternative means of synthesizing GFs are needed. Important GFs can be sourced from the genomes of different species. Similar sequences can be found by searching publicly available genome databases and synthesized or purchased from an available vendor. Recombinant DNA technology can then be used to insert sequences of interest into bacterial systems. Using this strategy, active GFs, such as FGF-2, IGFs, PDGF-BB, and TGF-β1 were effectively developed in Escherichia coli, and soluble GFs from cattle, chickens, and fish were produced [130].
CONCLUSION
GFs and hormones are important media components that regulate MSCs and improve cultured meat production. After binding to their receptors, these factors deliver the signals for growth, structural stability, cell-to-cell communication, proliferation, and differentiation. However, they are expensive media components, and thus, optimization and cheaper ways of synthesizing GFs and hormones are required. This review describes the importance of the regulatory roles played by different GFs (FGF-2, IGF-1, PDGF-BB, and TGF-1) and hormones (insulin, testosterone, glucocorticoids, dexamethasone, and thyroid hormones) during myogenesis and cultured meat production.