INTRODUCTION
As income levels increase, consumer diets become more varied, leading to a continuous rise in meat product consumption [1–3]. The market for diverse meat products is expanding alongside the growth in single-person households and a trend towards simpler diets. Various ingredients and food additives have been introduced to enhance the sensory and functional qualities of meat products [4,5]. Salt, a critical ingredient used in meat processing, improves myofibrillar protein solubility and enhances the functional properties of the meat, such as gel formation, water-holding capacity (WHC) , and emulsification [6]. It also contributes to flavor and texture enhancement and ensures microbiological safety [7].
The formation of meat protein gels through heating creates a three-dimensional network, primarily involving interactions between proteins, especially myosin [8]. The outer structure of the gel is mostly composed of insoluble proteins, while the inner structure is mainly composed of solubilized proteins [9]. The structure and properties of the gel are influenced by the degree of myosin cross-linking, which in turn is affected by protein solubility [10]. Post-slaughter adenosine triphosphate (ATP) depletion leads to the formation of a cross-linked actomyosin complex, reducing the solubility and gel-forming capability of the myofibrillar proteins [11]. Therefore, dissociating actomyosin is essential for enhancing the soluble myosin and actin content, thereby improving myofibrillar protein solubility. This improvement facilitates gel formation during heating by increasing water and lipid holding capacities [12]. As salt is added, it solubilizes myofibrillar proteins, boosting their solubility and contributing to the formation of a strong gel [13].
Gel properties are crucial for determining the digestibility and texture of meat products. When ingested, the bolus enters the stomach and is subjected to the acidic gastric fluid, leading to protein denaturation and structural unfolding [14,15]. Digestive enzymes in the gastrointestinal (GI) tract act on the exposed areas, resulting in protein degradation [16,17]. During this process, the extent of physicochemical denaturation and gel dissociation plays a vital role in exposing substrates to digestive enzymes [18]. At the supramolecular level, the extent of protein denaturation significantly affects the exposure of the cleaving sites, while the surface morphology or strength of the gel is also vital, as it influences the mechanical breakdown and enzyme movement during digestion [10]. Compact gel networks tend to slow the breakdown process, impeding protease access and movement [19].
Hence, variations in the NaCl content of meat products can significantly affect digestibility and sensory quality by altering the gel properties. This study sought to explore the physicochemical properties of gels in a sausage model with different NaCl concentrations (1.0%, 1.5%, and 2.0%, w/w) and to assess their digestibility during in vitro digestion. We hypothesize that protein solubility and actomyosin content vary with NaCl concentration, leading to differences in gel strength, protein denaturation, and, ultimately, digestibility.
MATERIALS AND METHODS
The pork picnic shoulder and back fat utilized in this experiment were acquired from a local market (Daejeon, Korea). The meat batters were formulated by incorporating 1.0%, 1.5%, or 2.0% (w/w) NaCl. For the preparation of the meat batters, ground pork meat (60%, w/w), back fat (20%, w/w), and ice (20%, w/w) were blended using a bowl cutter (C4 VV, Sirman SpA, Curtarolo, Italy). Sodium phosphate at a concentration of 0.3% (w/w) was then added and mixed for 1 min, followed by the addition of NaCl (1.0%, 1.5%, and 2.0%, w/w) and another min of mixing. Subsequently, isolated soybean powder (1.0%, w/w), sodium nitrite (0.01%, w/w), and L-ascorbic acid (0.02%, w/w) were incorporated and mixed for 2 min. Ice was added intermittently throughout the whole process to keep the temperature below 2°C. The resulting meat batters (45 g) were encased in stainless-steel containers with a 5 cm diameter, vacuum-packed (DWC-160, Duckwoo Machinery, Jangseon, Korea), stored at 4°C for 12 h, and then used to create pork gels as sausage models. The meat batters were thermally processed in a water bath at 80°C until the core temperature reached 75°C and then cooled at room temperature (25°C). The meat batters were prepared in three separate instances on an independent day for each treatment group, with three pork gels produced per batch (three treatments × three batches × three sausages); these batters were utilized as samples to analyze the quality characteristics.
To extract actomyosin from the meat batter, an aliquot (25 mL) of isolation buffer (0.1 M KCl, 2 mM MgCl2, and 1 mM ethylene glycol bis (2-aminoethyl ether)-N,N,N’,N’-tetraacetic acid dissolved in 10 mM potassium phosphate buffer, pH 7.0) was added to 2 g of meat batter and homogenized at 13,000 rpm for 15 s (T25 basic, IKA GmbH and Co. KG, Staufen, Germany). The homogenate was then centrifuged at 3,000×g for 10 min (1580R, LABOGENE, Lynge, Denmark) and the supernatant was discarded. This process was repeated by adding the same isolation buffer to the pellet, followed by homogenization and centrifugation to remove the supernatant. The pellet was then homogenized with 25 mL of extraction buffer (0.6 M KCl, 0.04 M NaHCO3, and 0.01 M Na2HCO3, pH 7.2) at 13,000 rpm (T25 basic), and the mixture was agitated at 4°C for 24 h. Afterwards, 20 mL of distilled water was mixed with 10 mL of the suspension, vortexed, and centrifuged to discard the supernatant. The final pellet was homogenized with 3 mL of KCl-Tris buffer (0.6 M KCl and 0.02 M Tris, pH 7.2) to obtain the actomyosin extract. The protein content of the extracts was determined using the Kjeldahl method (AOAC 928.08), and the actomyosin content of the meat batter was calculated as follows:
To assess total protein solubility, 1 g of meat batter was mixed with 20 mL of 0.05 M potassium phosphate buffer (pH 7.4) containing 0.55 M potassium iodide. The mixture was then homogenized at 13,000 rpm for 30 s (T25 basic). The homogenate was stirred at 4°C for 24 h (OS-2000, Jeio Tech, Daejeon, Korea), centrifuged at 3,000×g for 10 min (1580R), and the supernatant was filtered through filter paper (No. 4, Whatman, Maidstone, UK). The protein content of the filtrate was measured using a Varioskan LUX spectrophotometer (Thermo Fisher Scientific, Waltham, MA, USA) with a Bio-Rad assay kit (#5000006, Bio-Rad Laboratories, Richmond, CA, USA). A standard curve was generated using bovine serum albumin.
Cooking loss was determined by removing the cooked pork gels from the stainless-steel containers and weighing them after removing surface moisture. This was then compared to the pre-cooked weight of the meat batter. The calculation was as follows:
The texture of the pork gels was examined using a texture analyzer (Model A-XT2, Stable Micro Systems, Surrey, UK). The pork gels were cut into uniform pieces (2 × 2 × 1.5 cm). A compression probe with a diameter of 70 mm was affixed to the texture analyzer, and the samples underwent two cycles of 70% compression at a test speed of 2 mm/s to evaluate hardness and cohesiveness.
The protein digestibility in the pork gels was assessed using an in vitro digestion model. The in vitro digestion model was designed based on the method described by Minekus et al. [20]. All enzymes utilized in this study were sourced from Sigma-Aldrich (St. Louis, MO, USA). The oral fluid was adjusted to pH 7.0 after dissolving 75 unit/mL of α-amylase from Aspergillus oryzae, and the gastric fluid was adjusted to pH 3.0 after adding 2,000 unit/mL of pepsin from the porcine mucosa and 60 unit/mL of gastric lipase from Rhizopus oryzae. The GI fluid was prepared with 100 unit/mL of trypsin from porcine pancreas, 25 unit/mL of chymotrypsin from bovine pancreas, and 2,000 unit/mL of pancreatic lipase from porcine pancreas, while bile fluid, containing 10 mM bile extract from porcine pancreas, was prepared at pH 7.0.
Chopped pork gels were blended with the oral fluids at a 50:50 (v/v) ratio and stirred at 250 rpm for 2 min at 37°C. Simulated digestive fluids, composed of the stock solution, enzyme fluids, and distilled water, were added to the digestive fluids from the preceding compartment at a 50:50 (v/v) ratio. Each digestion step was conducted at 250 rpm for 2 h at 37°C. To exclude the protein content of the digestive enzymes from the analysis, blank samples were prepared by substituting the sample volume with an equal amount of distilled water and subjecting it to the same digestive conditions. The digesta samples were immediately frozen at −70°C post-digestion for subsequent analysis.
The 10% trichloroacetic acid (TCA)-soluble fraction was prepared by adding 10 mL of 12% TCA to 2 mL of digesta, followed by homogenization at 13,000 rpm for 30 s (T25 basic) and centrifugation at 3,000×g for 10 min. The supernatant was filtered through a filter paper (Whatman No.4) and reacted with o-phthalaldehyde (OPA). The OPA reagent, prepared fresh, consisted of 40 mg OPA dissolved in 1 mL methanol, 100 μL β-mercaptoethanol, 25 mM sodium tetraborate, and 2.5 mL of 20% sodium dodecyl sulfate (SDS), bringing the final volume to 50 mL with distilled water. An aliquot (150 μL) of the supernatant was mixed with 1 mL of the OPA reagent and reacted at 25°C for 2 min. The absorbance of the reaction mixture was measured at 340 nm using a plate reader (Varioskan LUX). A standard curve was generated using glycine, and the protein content was determined using the Kjeldahl method (AOAC, 928.08). The α-amino group content of the samples was expressed in mM/g.
The distribution of gel particles in the digesta was observed using fluorescence microscopy. The digesta samples were stained with Fast Green (1:100, v/v) and analyzed using an Olympus BX-53 microscope (Olympus, Optical, Tokyo, Japan) equipped with a fluorescence filter. Image analysis was performed using the Cell* image analysis software (Cell*, Sort Imaging System, Münster, Germany).
SDS-PAGE was carried out on a 12.5% polyacrylamide gel containing 30% acrylamide solution, 1.5 M Tris-HCl (pH 8.8), 0.5 M Tris-HCl (pH 6.8), 10% ammonium persulfate, and N,N,N’,N’-tetramethyl-ethylenediamine. Pork gels (1 g) were homogenized with 4 mL of 2% SDS in 0.01 M sodium phosphate buffer (pH 7.0). The homogenate was centrifuged at 1,500×g for 15 min (1580R) to collect the supernatant for electrophoresis. The pork gel and digesta samples, with a protein concentration of 2 mg/mL, were diluted with 2× sample buffer (EBA-1051, Elpis Biotech, Daejeon, Korea) and heated at 90°C for 10 min in a heating block before being immediately cooled on ice. The samples and a protein ladder (3453A, Takara Bio, Shiga, Japan) were loaded in volumes of 10 and 5 μL, respectively. Electrophoretic separation was performed using the PageRun system (AE-6,531 mPAGE, ATTO, Tokyo, Japan) at 20 mA for 120 min. The running buffer consisted of 25 mM Tris, 0.1% SDS, and 192 mM glycine. Protein bands in the gel were stained with Coomassie Brilliant Blue and then destained with a 10% acetic acid solution. The stained gels were scanned using a GS-710 densitometer (Bio-Rad Laboratories, Hercules, CA, USA) and analyzed using the Image Master 2D Platinum v5.0 software (GE Healthcare, formerly Amersham Biosciences, Seoul, Korea). Protein types were identified by comparing the molecular weight of the bands using the UniProt database (https://www.uniprot.org/).
This study was conducted in three replicates (three batches). Statistical analysis was performed using a mixed model with a randomized complete block design, treating the batch as a block and considering replications (batches) as random variables. The results are presented as the least-squares mean and the standard error of the least-squares means. The significance of the main effects was determined using Tukey’s multiple comparison test (p < 0.05). All statistical analyses were executed using the SAS software (version 9.4, SAS Institute, Cary, NC, USA).
RESULTS AND DISCUSSION
The effect of varying NaCl concentrations on the actomyosin content and protein solubility of the meat batter is shown in Fig. 1. The actomyosin content (Fig. 1A) was significantly lower at NaCl 2.0% (33.46%, p < 0.05) compared to NaCl 1.0% (42.19%). NaCl 2.0% also tended to have a lower actomyosin content than NaCl 1.5% (34.72%, p = 0.0504). Greene [21] reported that the addition of salt impacts the binding force between actin and myosin. Post-mortem, as the muscle pH decreases and the sarcoplasmic reticulum collapses, calcium ions (Ca2+) are liberated into the sarcoplasm [22]. These ions, upon interaction with troponin C located on the actin filaments, promote the exposure of the myosin-binding sites. This molecular mechanism enables repetitive cycles of muscle fiber contraction and relaxation, governed by the cyclical attachment and detachment of myosin to and from actin [12]. In the absence of ATP, actin and myosin remain bound, forming stable actomyosin complexes that are challenging to dissociate, reducing the solubility of myofibrillar proteins [22, 23]. Sellers [24] highlighted the role of aspartic and glutamic acids on the surface of the myosin motor head, serving as critical binding sites during actomyosin complex formation. The introduction of NaCl into meat batters leads to its dissociation into Na+ and Cl− ions; Na+ ions exhibit a high binding affinity for the COO− groups of aspartic and glutamic acid present on the protein surface [25]. This affinity may lead to the dissociation of the myosin head from actin, thereby decreasing the actomyosin content. The NaCl concentrations of 1.0%, 1.5%, and 2.0% (w/w) correspond to molar concentrations of 0.17, 0.26, and 0.34 M/L, respectively. According to the study of Greene [21], an increase in ionic strength from 0.12 M to 0.43 M significantly diminishes the binding capacity of heavy meromyosin to F-actin. Thus, an increase in NaCl concentration leads to a notable reduction in actomyosin content due to the reduced binding efficiency between actin and myosin.
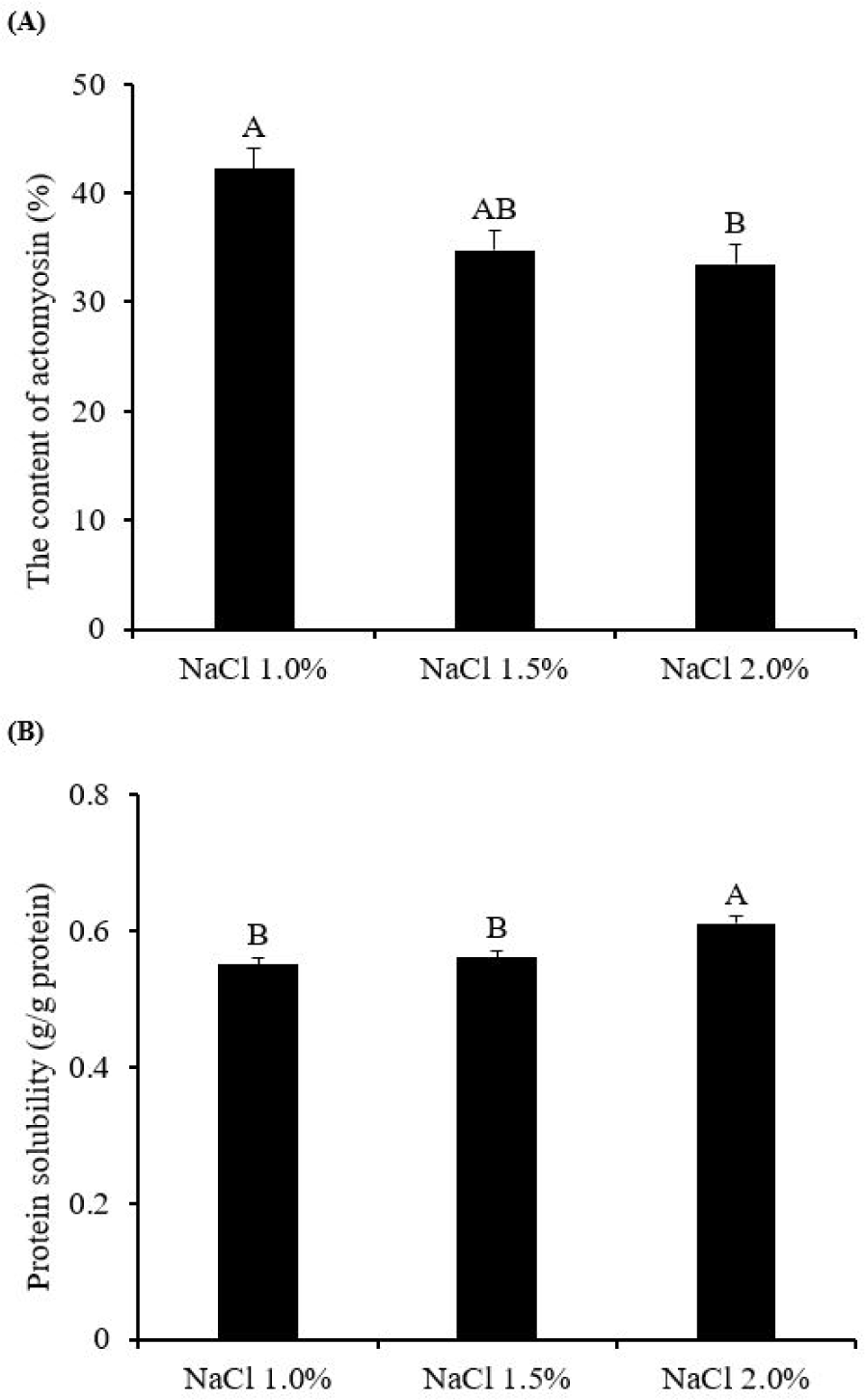
The solubilization process of myofibrillar proteins upon heating contributes to the formation of interfacial protein films, which are instrumental in trapping moisture and fat within the gel network [6]. This process underscores the importance of myofibrillar protein solubility as a critical functional property in the manufacturing of meat products [26]. As depicted in Fig. 1B, meat batter treated with NaCl 2.0% exhibited the highest protein solubility (0.61 g soluble protein/g total protein, p < 0.05) than NaCl 1.0% (0.55 g soluble protein/g total protein) and NaCl 1.5% (0.56 g soluble protein/g total protein), with no significant differences observed between the latter two concentrations (p > 0.05). This outcome can be attributed to the dissociated Na+ and Cl− ions in the meat batter forming ionic clouds that effectively shield the charged groups on the protein surface [7]. This shielding mechanism enhances the electrostatic repulsion between proteins and facilitates the rupture of ionic bonds, leading to the swelling and subsequent dissociation of thick myosin filaments [6,27]. As thick myosin filaments dissociate into myosin monomers, the overall structural stability of the myofibrillar proteins becomes diminished, increasing the amount of soluble protein [28]. Furthermore, the reduced actomyosin content observed at NaCl 2.0% may contribute to the enhanced total protein solubility by augmenting the content of soluble actin and myosin [9].
Taken together, these findings indicate that the meat batter treated with NaCl 2.0% exhibits the highest levels of actomyosin dissociation and protein solubility, suggesting that this particular concentration could potentially improve the gel-forming capacity of myofibrillar proteins, thereby influencing the properties of the resulting gel in a significant manner.
Fig. 2A shows the influence of NaCl concentration on the cooking loss of pork gels. The highest cooking loss was observed at NaCl 1.0% (9.07%, p < 0.05), followed by NaCl 1.5% (4.15%), and 2.0% (2.80%) (p < 0.05). Barbut [29] identified NaCl 1.5% as a critical threshold for enhancing the WHC in meat batters, a finding that is corroborated by our study, which demonstrated a significant increase in cooking loss at NaCl 1.0% compared to NaCl 1.5%. The observed higher protein solubility in NaCl 2.0% (Fig. 1B) suggests that a greater quantity of soluble proteins may enhance gel formation by more effectively trapping moisture within the gel structure [13].
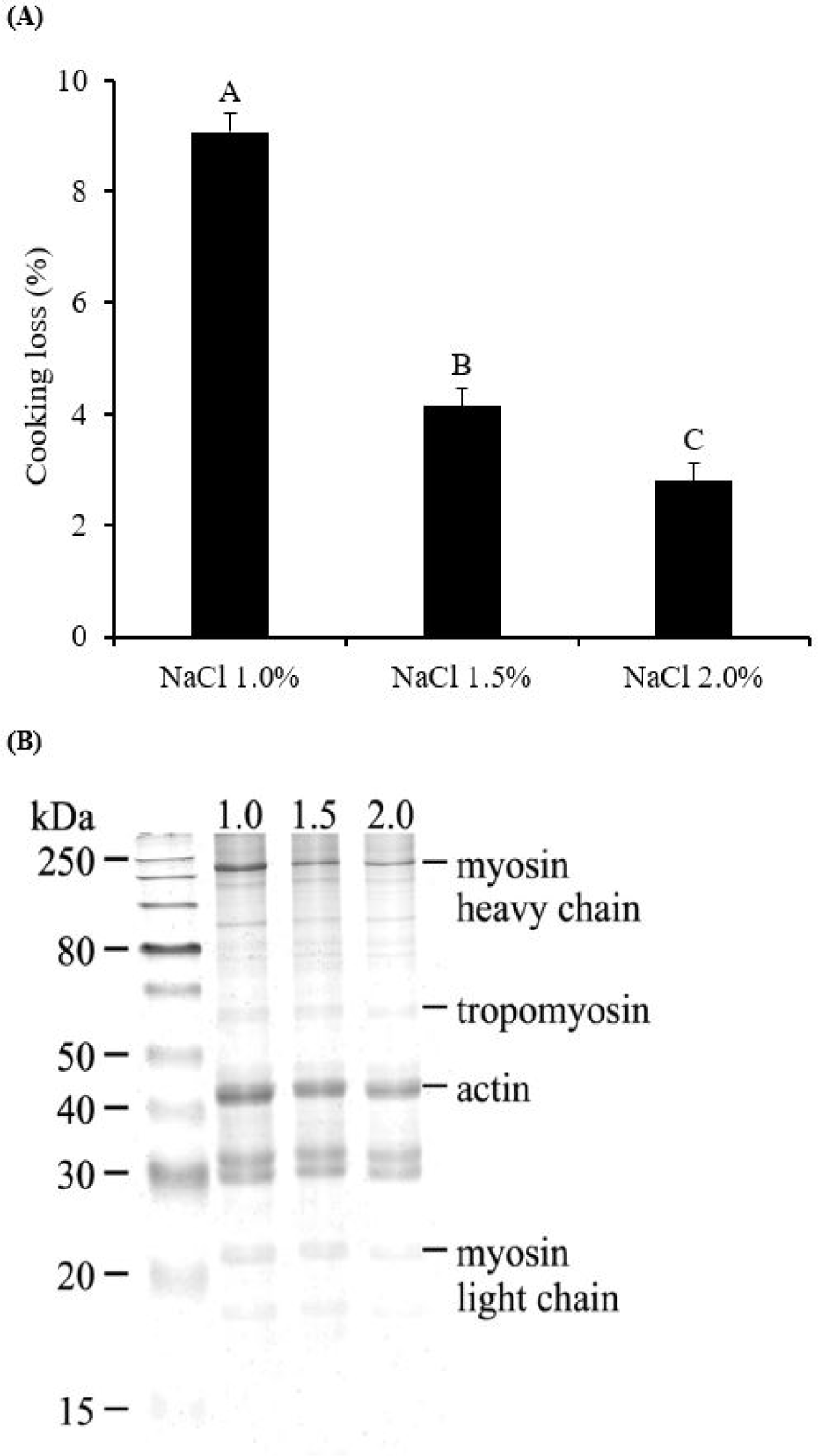
Additionally, the phenomenon of space expansion caused by charge shielding may also play a role in reducing cooking loss. The Na+ and Cl- ions bind to amino acids at opposite sites, leading to the swelling of the filament structure within muscle fibers, thereby increasing the capacity for water retention [30]. Consequently, NaCl 2.0%, characterized by a higher participation of soluble proteins in gel formation and an increased capacity to trap water, would likely result in the formation of a gel with enhanced moisture retention capabilities.
To further examine the textural properties of the pork gels, hardness and cohesiveness were assessed (Table 1). Hardness, indicative of the strength of the gel, was observed to be significantly higher in gels with NaCl 2.0% (102.47 N, p < 0.05) compared to those with NaCl 1.0% (76.19 N) and 1.5% (81.80 N), with no significant difference between the latter two (p > 0.05). This suggests that the gel formed with NaCl 2.0% possesses the highest structural integrity and resistance to deformation. Cohesiveness, reflecting the internal binding force and resilience of the gels, also exhibited the highest value at NaCl 2.0% (0.44), underscoring the enhanced structural cohesiveness of the gels and ability to resist breakdown under applied force. The lowest cohesiveness was observed at NaCl 1.0% (0.39). Although NaCl 1.5 % (0.40) showed no significant differences in cohesiveness compared to the NaCl 1.0% or 2.0% groups (p > 0.05), the textural properties of pork gels were affected by the concentrations of NaCl. These findings suggest that an increased NaCl concentration influences not only moisture retention but also the rigidity of the gels, making it an essential factor in the formulation of meat products with optimal sensory and functional characteristics.
Treatments | Hardness (N) | Cohesiveness |
---|---|---|
NaCl 1.0% | 76.19B | 0.39B |
NaCl 1.5% | 81.80B | 0.40AB |
NaCl 2.0% | 102.47A | 0.44A |
SEM | 2.560 | 0.014 |
Myosin, as a key protein involved in myofibrillar protein gelation, plays a crucial role during the heating process, where three-dimensional networks are formed through the aggregation of myosin heads and the crosslinking of tails [9,30]. Therefore, the solubilization of myofibrillar proteins, including myosin, becomes critical for the effective gelling of meat proteins. The salt-soluble nature of myofibrillar proteins implies that their solubility can be enhanced at higher NaCl concentrations, as observed with NaCl 2.0% [6], compared to lower concentrations. This enhanced solubility facilitates the formation of interfacial films of proteins, which play a crucial role in capturing water and fat globules within the gel network, contributing to reduced cooking loss and the formation of a gel with superior strength due to the improved emulsifying capacity [26]. Sun and Holley [9] highlighted that a NaCl concentration of 0.3 M (2%‒3% NaCl) is necessary to form a myofibrillar protein gel with high structural strength. This underscores the hypothesis of our study that the addition of NaCl 2.0% to the meat batter leads to the formation of a gel with the highest strength during heating, attributed to the optimal extraction of salt-soluble proteins.
Investigating the digestive behaviors of proteins involved a multipronged approach. We analyzed α-amino group content, observed fluorescence microscopic images of the digesta, and examined the electrophoretograms of gastric and GI digesta from samples after in vitro digestion (Figs. 3, 4, and 5, respectively).
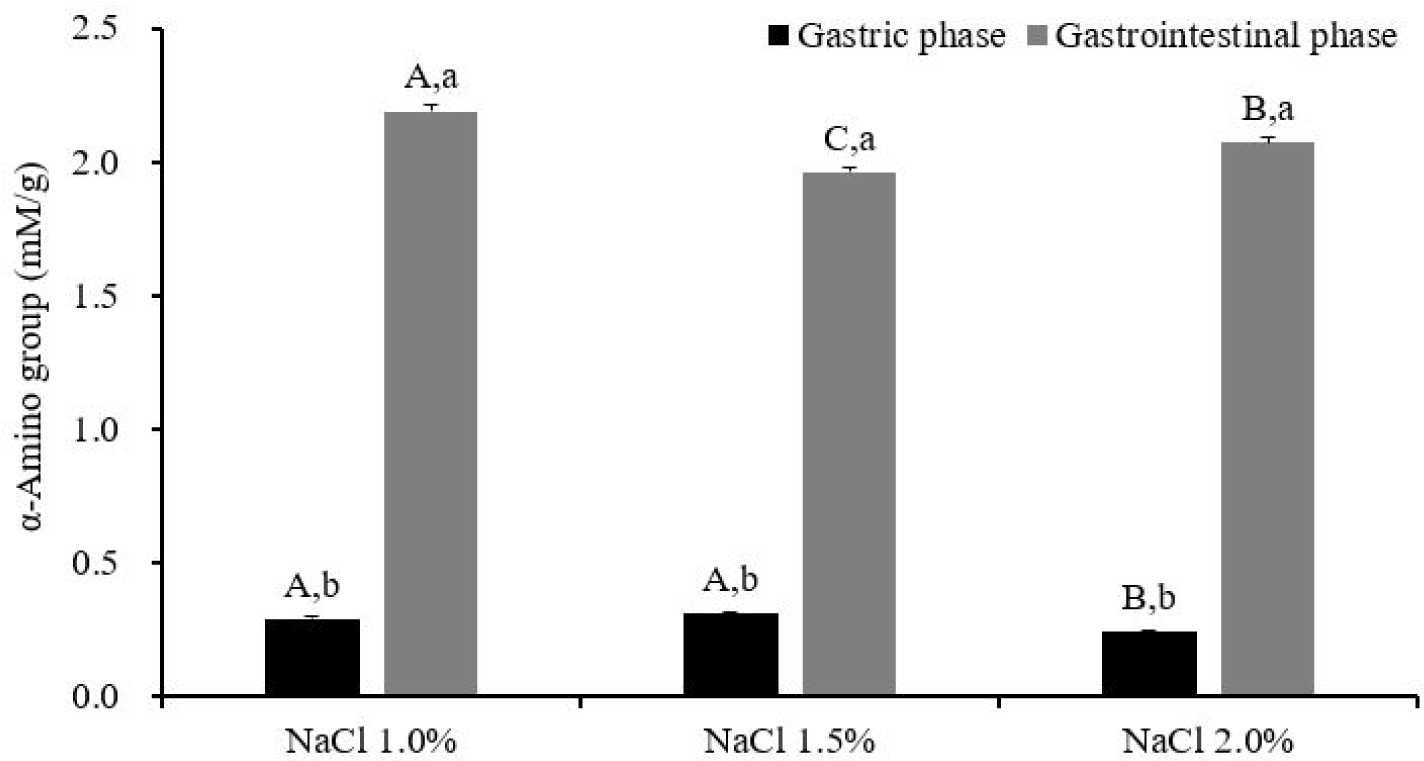
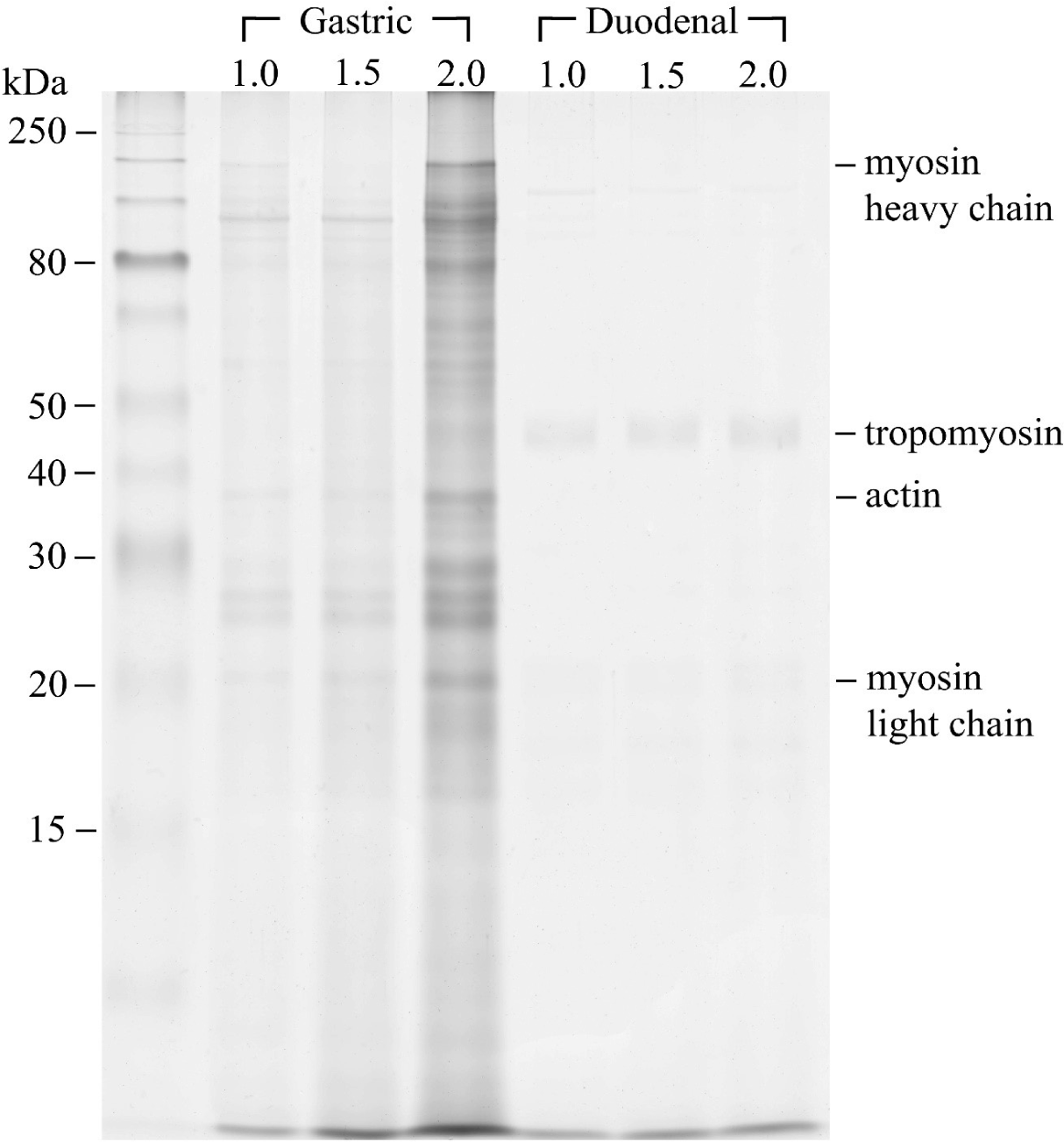
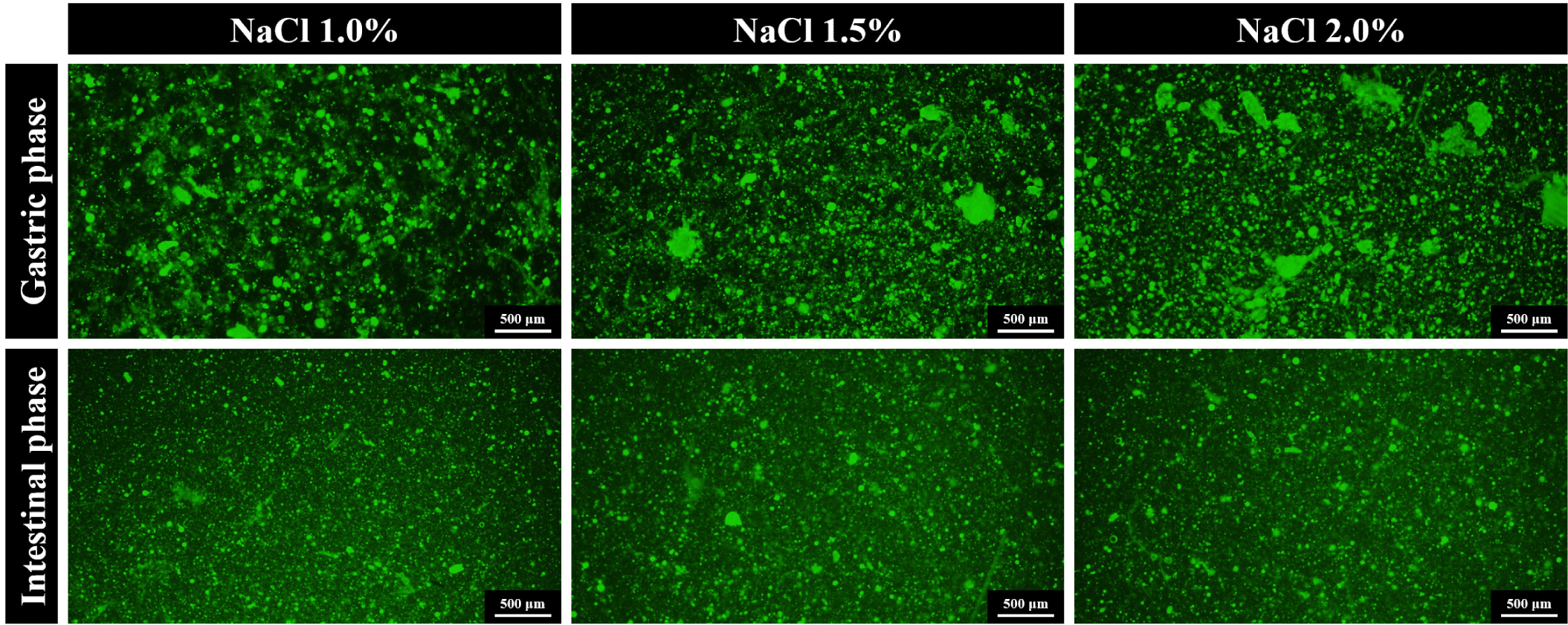
Gastric phase: α-Amino group content in the gastric digesta was found to be lowest in the NaCl 2.0% treatment (0.24 mM/g, p < 0.05), followed by NaCl 1.5% (0.31 mM/g) and 1.0% (0.29 mM/g), indicating a slower pepsinolysis rate for NaCl 2.0% during gastric digestion (Fig. 3). The SDS-PAGE electrophoretogram of the gastric digesta (Fig. 4) revealed the near disappearance of protein bands that were originally present in the lanes (Fig. 2B) for the NaCl 1.0% and 1.5% treatments, while some bands persisted at 20‒45 kDa in the NaCl 2.0% treatment. These bands were attributable to tropomyosin (32 kDa), actin (42 kDa), and the myosin light chain (223 kDa). These results implied that the protein digestibility of NaCl 2.0% treatment was lower than those of NaCl 1.0% and 1.5% treatments. Fluorescence microscopy showed a uniform distribution of small gel particles in the digesta of NaCl 1.0% (Fig. 5). However, in the case of NaCl 2.0%, un-dissociated and aggregated particles were observed, suggesting insufficient dissociation of gel particles during gastric digestion in this treatment. The high gel strength of NaCl 2.0% likely contributed to the lowest release of α-amino groups during this phase. Protein digestion in the gastric phase is predominantly facilitated by pepsin, with its cleavage specificity for phenylalanine, tyrosine, tryptophan, and leucine at position P1 [31], contrasting with the extensive digestion by trypsin and chymotrypsin in the small intestine phase [10]. The limited degradation capacity of pepsin in the gastric phase and the mechanical destruction of gels under acidic conditions highlight the necessity for the penetration and diffusion of acidic gastric fluid and enzymes after the physical dissociation of the gels for effective gastric pepsinolysis [17,19]. The slower dissociation of gels during digestion results in a reduced surface area for enzyme activity [32]. Thus, denser and less dissociated gels due to the slower dissociation of the NaCl 2.0% gel exhibit the lowest α-amino group content. This observation aligns with Dong et al. [19], who reported the lowest digestibility of whey protein gels containing a high NaCl concentration due to delayed gel dissociation during gastric digestion.
Sayd et al. [33,34] reported that undigested protein bands were identified after 180 min of gastric digestion, and the peptides originating from myofibrillar proteins could be identified in the digesta of small intestinal phase. Lee et al. [14] observed that actin and myosin light chain bands remained in digesta electrophoretogram, especially after gastric digestion. These were similar in this study (Fig. 4). The proteolytic susceptibility of myosin to the actions of trypsin and chymotrypsin, contrasted with actin due to its robust core, resulting in the production of large fragments with a molecular weight of approximately 33 kDa [35], explaining the presence of the remaining bands in gastric digesta (Fig. 4).
GI phase: After the GI digestion, NaCl 1.0% retained its highest content of the α-amino group content with 2.19 mM/g (p < 0.05). Permeability within the gel structure is important for the action of digestive enzymes. Dong et al. [19] noted that digestive enzyme diffusion is generally more extensive in a loose gel structure with a large mesh size, implying that NaCl 1.0% underwent the most rapid digestion in both the gastric and GI phases due to its lower gel strength. Conversely, NaCl 1.5% (1.96 mM/g) exhibited the lowest content of α-amino groups, followed by NaCl 2.0% (2.07 mM/g), indicating that NaCl 1.5%, with intermediate gel strength, underwent higher protein degradation and faster gel dissociation than NaCl 2.0% in the gastric phase, but resulted in less protein degradation than NaCl 2.0% during the GI phase. This finding suggests that the degree of protein denaturation is a critical factor for digestive accessibility during the GI phase. Unlike the gastric phase, where pepsin is the primary proteolytic enzyme, GI digestion involves trypsin and chymotrypsin. Trypsin specifically targets peptide bonds at the C-terminal of lysine and arginine residues, while chymotrypsin preferentially cleaves at tryptophan, tyrosine, and phenylalanine residues at position P1 [31]. Thus, the synergetic actions of trypsin and chymotrypsin lead to extensive protein cleavage. In addition, the decomposition of the gel structure already occurs during the gastric digestion, and complete destruction of the gel matrix containing fat globules additionally occurs in the small intestinal phase with the emulsification by bile acids. Thus, since the mechanical destruction of gel particles will occur in the small intestinal phase, the degree of exposure of the cleaving sites of trypsin and chymotrypsin depending on the protein structure may be more important for protein digestibility than the effect of gel particle rigidity. The presence of Na+ and Cl− ions disrupts the hydration layer on the protein surface, exposing hydrophobic amino acids within the structure and leading to the denaturation of myofibrillar proteins [36]. Increased NaCl concentrations can induce greater protein denaturation, including the exposure of non-polar amino acids and alterations in secondary structure through hydrogen bond disruption [37]. Excessive denaturation may interfere with gel formation or digestive susceptibility due to protein aggregation, whereas partial denaturation may enhance enzyme accessibility [10].
We hypothesized that the NaCl 2.0% gel, potentially exhibiting the greatest degree of protein denaturation, would demonstrate higher digestive susceptibility to trypsin and chymotrypsin in the GI phase. Contrary to the gastric phase, fluorescence microscopy images in the GI phase showed a uniform distribution of small particles across all GI digesta (Fig. 5), with the electrophoretogram indicating the disappearance of bands present in the NaCl 2.0% lane after GI digestion (Fig. 4). This observation aligns with the findings of Lee et al. [17], who reported that egg white protein gel cooked at 95°C exhibited the slowest gastric digestion due to minimal gel dissociation but showed the highest GI digestibility due to the greatest degree of protein denaturation.
CONCLUSION
This study investigated the impact of various NaCl concentrations on pork sausage models, focusing on physicochemical characteristics and in vitro protein digestion. We found that a NaCl 2.0% concentration significantly altered the actomyosin content, increased protein solubility, and resulted in the highest hardness and cohesiveness of pork gel.
After in vitro digestion of the pork gels, the α-amino groups in the 10% TCA-soluble fraction of the gastric digesta were higher in NaCl 1.0% and 1.5% than in NaCl 2.0%, as confirmed by the electrophoretogram of gastric digesta of NaCl 2.0% displaying the clearest and most numerous remained protein bands. Fluorescence microscopic images of the gastric digesta revealed large and undissociated gel particles in NaCl 2.0%, while after GI digestion, all treatments showed uniform distribution and small particle sizes. NaCl 1.0% pork gel retained the highest content of α-amino groups after GI digestion, followed by NaCl 2.0% and 1.5%, as NaCl 1.5% exhibited the lowest α-amino group release.
In conclusion, our findings confirm that gel properties and in vitro protein digestive behavior vary depending on NaCl concentration in pork sausages. Therefore, to develop meat products with superior sensory and nutritional qualities, the impact of gel physicochemical properties on protein digestion, particularly for NaCl levels, should be considered.