INTRODUCTION
The Qinghai-Tibetan Plateau (QTP), characterized by low temperature and hypobaric hypoxia, is the highest plateau in the world, with an average altitude > 4,000 m above sea level. Species are facing strong selection pressure to adapt to inhospitable high-altitude environments [1]. The yak (Bos grunniens) is an important domesticated ruminant. It is the only large mammal inhabiting the QTP and is an iconic symbol of the QTP [2]. Yaks living at high altitudes more than 7,000 years, and must adapt to the stress of decreased oxygen availability [3]. Yaks have numerous special morphological and physiological mechanisms for life at high altitudes, e.g., blunted hypoxic pulmonary vasoconstriction [4], increased foraging ability [5], enhanced glucose uptake and aerobic respiration [6], and improved bioenergy metabolism than mammals living in the plains [7]. Genome analysis identified an expansion in yak of gene families related to sensory perception and energy metabolism comparied with cattle [8], and differentially expressed miRNAs have also been found to be enriched in hypoxia-related pathways [9]. In addition, to reduce the risk of infection and disease, the activation of innate immunity was higher in yaks than in other cattle [10]. These findings partially reveal the adaptive mechanisms of yaks due to natural selection in a high-altitude and hypoxic environment, but few investigations have focused on the role of metabolites.
High-altitude hypoxia continuously affects the physical performance of people and animals [11]. Survival in high-altitude hypoxia requires a profound adaptive shift in metabolic processes [12]. In addition, hypoxia is related to homeostasis and the metabolic rate in adult tissues [13]. Organic metabolites are the reactants, intermediates or products of enzymatic reactions and represent the final products of cellular processes. The trend of contemporary scientific development is to follow systems biology. Investigation into the metabolome in response to genetic modification or physiological stimulus is a part of systems biology [14]. Identifying metabolic pathways has the potential to improve the understanding of physiological mechanisms [15]. The metabolome can reveal total metabolic profile changes in biological phenotypes and silent phenotypes [16]. In this study, the serum metabolites of yaks (B. grunniens), yellow cattle (Bos taurus) and China Holstein dairy cows (Bos taurus) were analyzed using a nontargeted metabolomics approach based on ultra-performance liquid chromatography-quadrupole time-of-flight mass spectrometry (UPLC-Q-TOF-MS). Comparing yaks, yellow cattle and China Holstein dairy cows may contribute to understanding evolutionary adaptation and provide meaningful data for survival at high altitudes.
MATERIALS AND METHODS
Blood samples were collected between 9:00 and 10:00 am by jugular venous puncture using vacuum tubes from 12 white yaks (Qilian Township, 37°41’6”N, 102°26’24”E, altitude: 3,600 m), 12 local yellow cattle (Tanshanling Town, 37°4’38”N, 102°24’14”E, altitude: 2,200 m), and 12 China Holstein dairy cows (Anyuan Town, 37°8’24”N, 102°37’48”E, altitude: 1,700 m) in Tianzhu County on the edge of QTP, Gansu Province, China (Fig. 1). After the blood was left to stand for 30 min, it was centrifuged at 1,000 ×g for 5 min at 4°C. Then, the serum was extracted, immediately frozen in liquid nitrogen and stored until analysis was carried out. All animals are female, and about three years old. Yaks and yellow cattle graze the natural grassland throughout the year without supplementary feed and housing. Holstein dairy cows (milk production: 27.1 ± 0.85 kg/day, parity: 2, days in milk [DIM]: 91.6 ± 7.5 days) were fed the total mixed rations (TMR) diets ad libitum, the basal diet was formulated based on the Feeding Standards of Dairy Cattle in China. The three species had similar physical characteristics, and the characteristics enrolled yak, cattle, and Holstein dairy cows are shown in Table 1. The three pasture sites are traditionally used by local herders for grazing, with similar environment and climatic conditions (temperature: 19.2 ± 1.1°C, relative humidity: 65.0 ± 2.2%), except altitude. In order to minimize the controlling variables of feeding and environmental factors among the three species, the blood was collected in August, 2021. The animal experiment was approved, and the animals received humane care according to the Ethical Committee rules of Lanzhou University (RIB21110301).
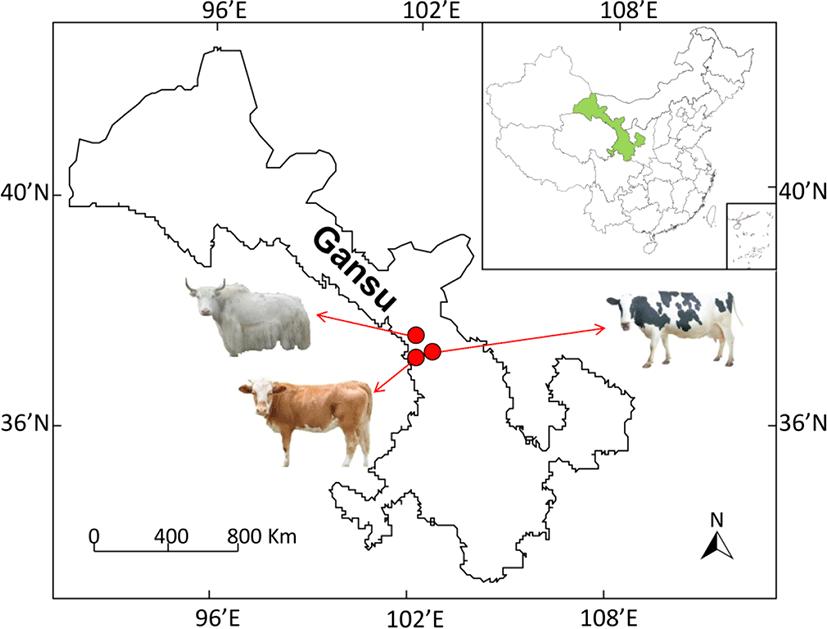
The collected samples were thawed at 4°C, and 100 μL of sample was mixed with 400 μL of precooled methanol/acetonitrile (1:1, v/v). It was incubated at room temperature for 10 min and then centrifuged. The supernatants were collected, dried, and then resuspended in 30 μL water/acetonitrile (98:2, v/v) for MS analysis.
First, a UPLC system (SCIEX, Framingham, MA, USA) was used for chromatographic separations. Reversed-phase separation was performed using an ACQUITY UPLC T3 column. Solvent A (ultrapure water, 0.1% formic acid) and solvent B (acetonitrile, 0.1% formic acid) comprised the mobile phase.
The metabolites were detected using a tandem mass spectrometer (TripleTOF5600plus, SCIEX). The details of the Q-TOF mass spectrometry conditions were based on our previous publication [17].
LC−MS raw data files were processed by the CAMERA and XCMS packages of R software. Retention time (RT) and m/z data were used to identify each ion. The metabolites were annotated using the human metabolome database (HMDB) database and Kyoto Encyclopedia of Genes and Genomes (KEGG) analysis. MetaX was used to further preprocess the intensity of the peak data. The “50% rule” was applied to remove the systematic bias or technical variation by normalizing the data according to our previous publication [17], and the results showed a normal distribution after normalization processing. Outlier detection and batch effects were evaluated by principal component analysis (PCA). False discovery rate (FDR) and supervised partial least squares-discriminant analysis (PLS-DA) were conducted to adjust the p value. The important features were selected based on a variable importance in the projection (VIP) cutoff value of 1.0.
The levels of interleukin-2 (IL-2), interleukin-6 (IL-6) and tumor necrosis factor-α (TNF-α) in serum were determined using ELISA kits. The levels of malondialdehyde (MDA), total antioxidant capacity (T-AOC), and glutathione peroxidase (GSH-Px) in serum were measured by chemical colorimetry. All kits were purchased from Nanjing Jiancheng Bioengineering Institute (Nanjing, China). The detailed principles and methods for the detection of MDA, T-AOC and GSH-Px have been described in our previous publication [18].
RESULTS
Box plots were used to analyze the identified serum metabolites in yaks, yellow cattle and dairy cows. All samples showed a similar range of metabolite levels (Fig. 2A). Serum metabolomic analysis was used to determine whether the metabolic profiles of yaks are separable from those of dairy cows and yellow cattle, we used PCA for visualization. Based on the serum metabolic profiles, the score plots of the PCA model discriminating yaks, dairy cows and yellow cattle are presented in Figs. 2B and C. PCA showed that the positive mode of the total variance data was 41.23%, represented by the first two principal components (Fig. 2A), and the negative mode was 52.17% (Fig. 2B). The plot revealed that the serum metabolic profiles of yellow cattle were closely related to those of dairy cows and were not obviously changed. However, the profiles of yaks showed a clear separation trend from those of and yellow cattle and dairy cows.
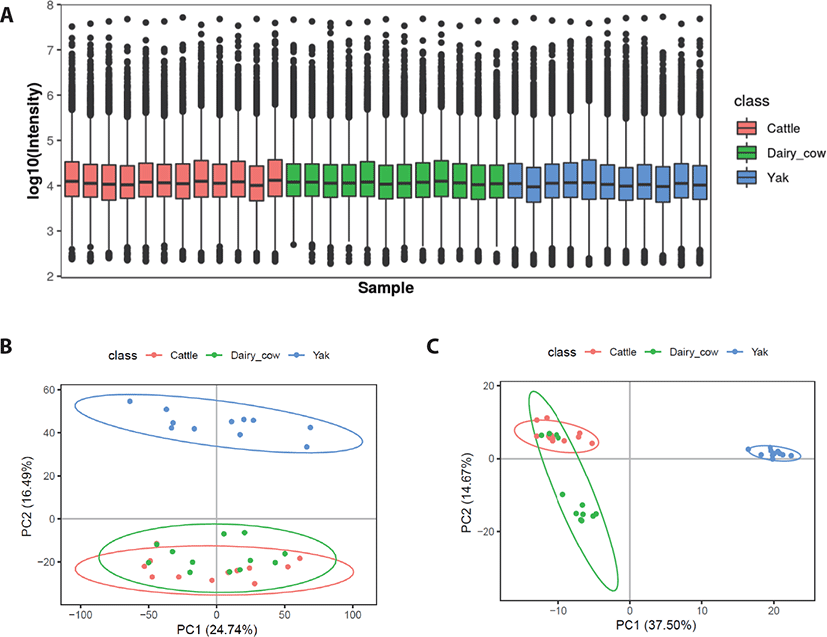
From the 1,815 detected metabolites, we investigated 63 differentially expressed metabolites (Table 2), including L-glutamine, L-glutamic acid, α-linolenic acid, tauroursodeoxycholic acid, and LysoPC (ratio ≥ 5.0 or ≤ 0.2, p < 0.01 and VIP ≥ 1). The relative concentrations of 23 metabolites were significantly higher, while 30 were significantly lower in yaks than in yellow cattle (Table 2). The relative concentrations of 11 metabolites were significantly higher and 15 were significantly lower in yaks than in dairy cows. The relative concentrations of 11 metabolites were significantly lower in yellow cattle than in dairy cows. These metabolites were carbohydrates, amino acids, lipids and their metabolites, suggesting that these metabolic pathways were different among yaks, yellow cattle, and dairy cows. Furthermore, metabolic pathways were significantly different between yaks and yellow cattle and between yaks and dairy cows, but there were similarities between yellow cattle and dairy cows based on the very few differential metabolic profiles (Table 2).
KEGG analysis was used to predict metabolic pathways for all differential metabolites. Fig. 3 shows the functional enrichment of the top 5 different pathways. The most enriched functional pathways among yak, yellow cattle and dairy cow belonged to metabolic pathways: amino acid metabolism (e.g., phenylalanine, arginine, proline, glycine, valine, leucine, isoleucine and glutamine), phospholipid metabolism (lysophosphatidylcholines [LysoPCs]), and fatty acid metabolism (arachidonic acid metabolism, α-linolenic acid and linolenic acid metabolism).
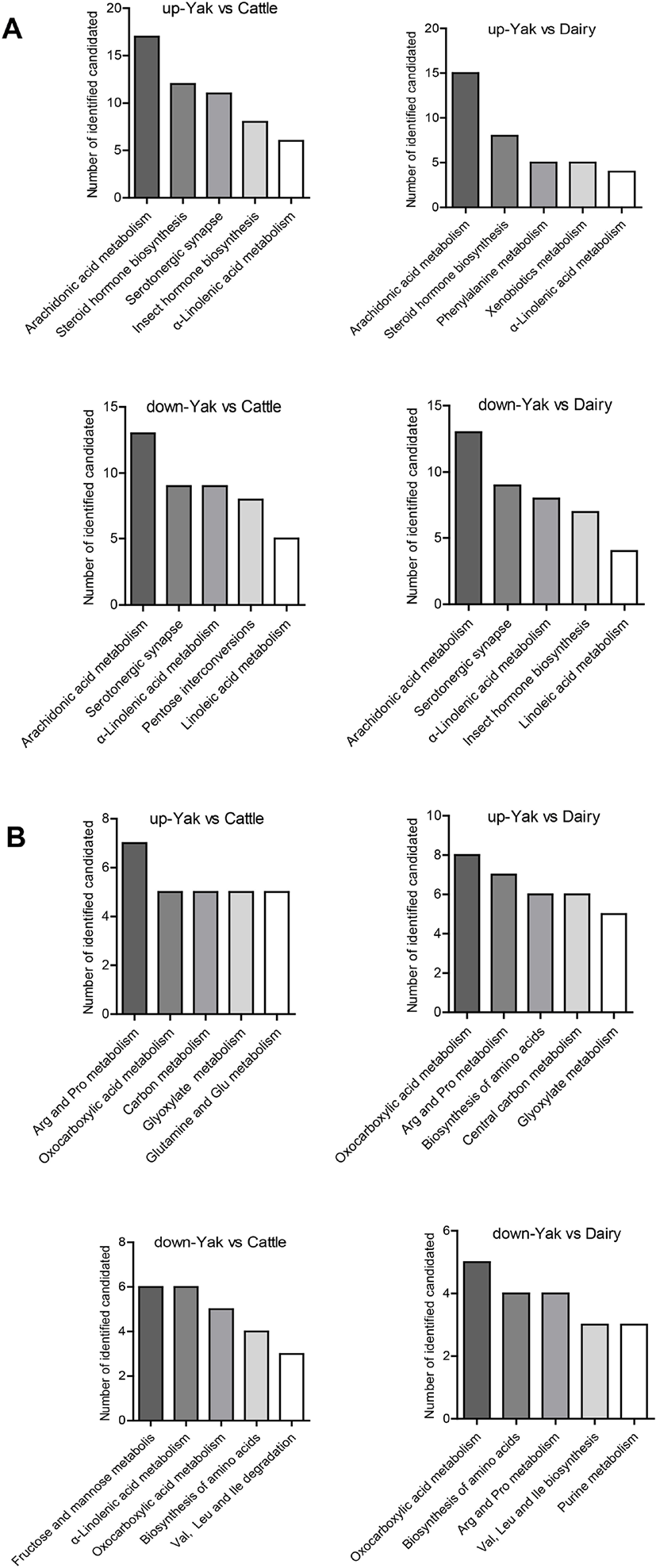
The levels of the inflammatory cytokines IL-2, IL-6, and TNF-α in yak serum were significantly higher than those in yellow cattle and dairy cows (p < 0.05 or p < 0.01) (Figs. 4A, B and C). Oxidative stress is an imbalance of reactive oxygen species (ROS) generation and elimination. High altitude-associated hypobaric hypoxia stress induces ROS production [19]. To determine if the elevated peripheral inflammatory cytokines were accompanied by reactive oxygen production or oxidative damcage [20], serum levels of MDA, T-AOC and GSH-Px were measured by chemical colorimetry. The results showed that there was no significant differences in MDA, T-AOC and GSH-Px levels among yaks, yellow cattle and dairy cows (p > 0.05) (Figs. 4D, E, and F).
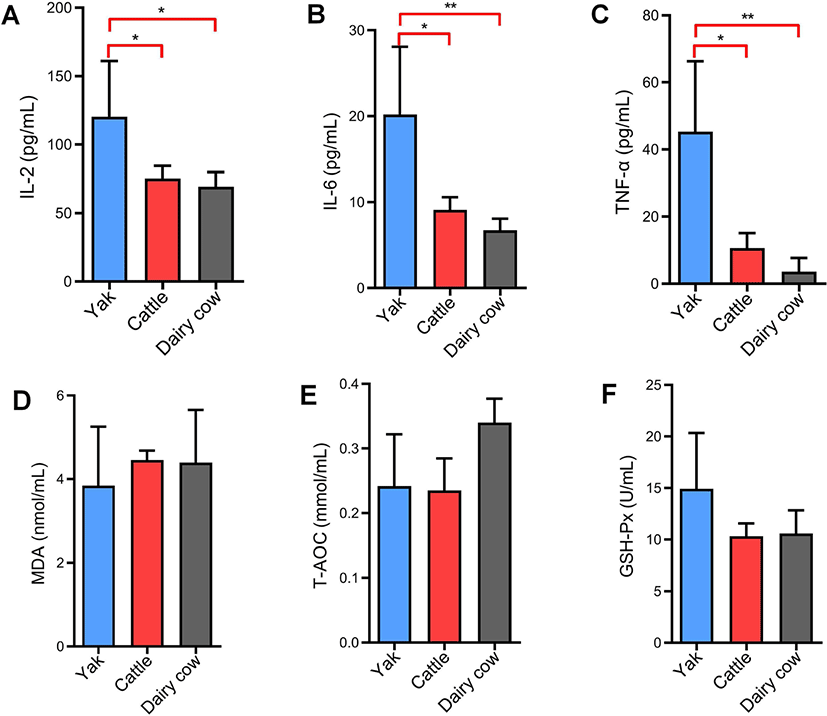
DISCUSSION
Yaks are an iconic symbol of QTP and can be used as a model to elucidate the mechanisms of hypoxia adaptation. The three specises of yak, yellow cattle and Holstein dairy cow belong to subtribe Bovina [21]. Holstein and yellow cattle should be probably separated from yak about 4.4 to 5.3 million years ago [10,22]. Systems biology is the trend of contemporary scientific development [23]. Comparative transcriptome sequencing revealed that the innate immunity were more activated in yak lung than low-altitude cattle (Sanjiang and Holstein cattle) [10,24]. Proteomics of skeletal muscle mitochondria showed that the significantly affected pathway in yaks and cattle was oxidative phosphorylation [7]. Identification of metabolic pathways using metabolomics comparisons between closely related species has the potential to provide insights into the basis of mammalian divergence and adaptation. To understand differences in the global metabolic profiles and relevant metabolic pathways of yaks, yellow cattle and dairy cows during acclimatization to high altitude, we utilized UPLC-Q-TOF-MS to determine the serum metabolite profiles of the three breeds.
We detected a clear separation trend between yaks and yellow cattle and dairy cows. A total of 63 different metabolites were obtained in serum. An integrative view plot of the metabolic changes among white yaks, yellow cattle and dairy cows was prepared (Fig. 3). The major perturbed metabolic patterns and plausible pathways are involved in amino acid metabolism, phospholipid metabolism, and fatty acid metabolism, which are associated with hypobaric hypoxia.
Glutamine is a key metabolite in the alanine, aspartate and glutamate metabolism pathways [25]. A previous study reported that high-altitude exposure leads to lower glutamate levels due to decreased activity of glutamine synthetase [26]. In contrast, we found significantly elevated levels of glutamic acid and glucogenic amino acids that produce pyruvic acid, α-ketoglutaric acid, and oxaloacetic acid in the serum of yaks in comparison with yellow cattle and dairy cows [27] (Table 2). The results of this study indicate that yaks have the highest levels of protein catabolism and amino acid mobilization. Therefore, the mobilization of yak muscle protein may be a metabolic adaptation to hypobaric hypoxia. Pathway analysis also showed improved energy metabolism and promoted acclimatization to high altitude by increasing the metabolism of phenylalanine, arginine, proline and glutamine to meet the energy requirements in yaks (Fig. 3). This result is consistent with the previous finding that hypobaric hypoxia exposure can enhance glucose and amino acid metabolism [28].
Phospholipids play a role as a cellular bilayer with membrane proteins, and they are involved in the maintenance of hepatic lipid metabolism [29]. Our results show that almost all LysoPCs, including LysoPC (18:0), LysoPC (16:0), LysoPC (18:1), LysoPC (22:6) and LysoPC (22:4), were markedly increased in yaks compared with yellow cattle and dairy cows (Table 2). LysoPCs participate in the inflammatory response by mediating cell signaling pathways in monocytes and macrophages [30,31]. To verify the increased LysoPCs, the serum levels of cytokines IL-2, IL-6, and TNF-α were detected. The results showed that the levels of IL-2, IL-6, and TNF-α were significantly higher in yaks than in cattle and dairy cows (Figs. 4A, B, and C), which is consistent with the increased LysoPCs. Environmental factors such as hypobaric hypoxia, cold and UV exposure at high altitude can suppress the immune system [32]. Tumor necrosis factors and interleukins can mediate innate immunity signaling. Xin et al. [10] reported that the immune system was more activated and the genes related to immune were up-regulated in yak compared with Sanjiang and Holstein cattle [10]. A significant elevation of LysoPCs and cytokines (IL-2, IL-6, and TNF-α) might be responsible for yaks being more tolerant to hypoxia at high altitudes than yellow cattle and dairy cows by activating innate immunity system.
Hypoxia is associated with an increase in the generation of reactive oxygen species (ROS), and an excessive load of ROS generated may result in cell injury and dysfunction [33]. Excessive ROS can lead to lipid peroxidation, MDA can reflect the level of lipid peroxidation. ROS are balanced by natural antioxidant compounds such as GSH-Px, superoxide dismutase (SOD) and catalase (CAT) [34]. We found that serum MDA levels, T-AOC and GSH-Px activity were not significantly changed in yak in comparison with yellow cattle and Holstein dairy cows (Figs. 4D, E, and F), based on they had similar physical characteristics (Table 1). The results demonstrate that yaks adapt to hypoxia-induced oxidative stress at high altitudes do not by increasing antioxidant enzyme levels. Free fatty acids (FFAs) are risk factors for cardiovascular diseases and are closely related to metabolic syndromes [35]. FFAs are significant sources of ROS [36], mainly through the activation of NADPH oxidase [37]. There was a dose-dependent increase in ROS in monocytes exposed to FFAs [38]. Polyunsaturated fatty acids (PUFAs) are a favorable target for ROS [39]. Oxidative breakdown of PUFAs may affect lipid metabolism and the expression of genes and proteins related to cell differentiation [40]. α-Linolenic acid and linoleic acid are PUFAs. Linoleic acid contains unsaturated double bonds that are highly vulnerable to ROS [41], and has been linked to red blood cell damage by promoting redox reactions [42]. The ROS production was greater in bovine mammary epithelia cells treated with linoleic acid and α-linolenic acid [43]. Arachidonic acid has been demonstrated to promote inflammatory responses by activating the mitogen-activated protein kinase (MAPK) and c-Jun N-terminal kinases (JNK) pathways by increasing TNF-α levels [44,45]. Arachidonic acid-derived metabolites also can propagate inflammation and oxidative stress [46]. Arachidonic acid suppressed the cell growth of hepatic cells by dose‐dependently inducing the production of ROS [47]. In the present work, FFAs (α-linolenic acid, linoleic acid and arachidonic acid) were significantly decreased in yaks compared with yellow cattle and Holstein dairy cows (Table 2). We speculate that yaks can decrease the level of FFAs (α-linolenic acid, linoleic acid and arachidonic acid) in serum induced by hypoxia and that the decreased FFAs can attenuate cell injury and hypoxia dysfunction by inhibiting oxidative stress.
CONCLUSION
A clear separation trend between the serum metabolic profiles of yaks and yellow cattle and dairy cows was demonstrated by PCA. In addition, a total of 63 differentially expressed metabolites were identified among the three species. Functional analysis revealed that differentially expressed metabolites were related to the innate immune activation (elevation of LysoPCs and cytokines), oxidative stress-related metabolism (arachidonic acid metabolism, α-linolenic acid metabolism, and linoleic acid metabolism) and energy metabolism (fatty acid metabolism and amino acid metabolism) in yaks, which indicates the important roles of metabolites in high-altitude adaptation in yaks.