INTRODUCTION
Population aging is a worldwide phenomenon. According to the World Health Organization (WHO), there will be an increase in the global aging population from 12% to 22% between 2015 and 2050 [1]. Most European countries have already entered an aging society, and their population groups of older adults (defined as 65 and over) are gradually increasing [1]. The Japanese population is also rapidly aging; the Organisation for Economic Co-operation and Development (OECD) survey in 2015 showed that Japan had the most aged society globally, with 26.3%. Germany, Greece, and Italy have also entered a super-aged society, with an aging rate exceeding 20% [2]. In 2015, the US also entered an aging society, with older adults accounting for 14.9% of the total population [3]. South Korea has an aging rate of 16.5%, indicating that it, too, has already entered an aging society [4]. According to OECD forecasts, most OECD member countries will become super-aged societies by 2030 [5]. Thus, it is imperative to address the health status of this aging population.
Proper nutrition, or healthy eating, has been linked to self-sufficiency and independent living, a decreased risk of chronic diseases, notably obesity, diabetes, coronary heart disease, and some cancers, in addition to enhanced quality of life among older adults [6]. Across the globe, nutrition and quality food standards for older adults are being established, and many companies are developing foods targeting this segment of the population. The U.S. Food and Drug Administration (FDA) categorizes such foods as medical foods designed for nourishment during physical, physiological, and pathological challenges, such as allergies, diseases, and recovery.
Meat and meat products are good protein sources for humans. Meat proteins are well-balanced in amino acids and contain all the essential amino acids [7,8]. However, older adults often avoid consuming meat products due to difficulties with digestion or chewing. Therefore, this review provides basic information for the improved protein digestibility by comprising results on the processing methods for improving meat digestion and their mechanism.
PROPOSAL FOR THE IMPROVEMENT OF PROTEIN DIGESTIBILITY IN OLDER ADULTS
Aging can lead to natural teeth loss, decreased masticatory function, dysphagia, decreased sensations (such as sight, smell, and taste), indigestion, poor diet, and depression, all of which are known to be intimately associated with reduced dietary intake or malnutrition in older adults [9]. Physiological changes in the aging gastrointestinal tract (GIT) contribute to the development of malnutrition, which, in turn, increases the risks for the development of chronic disabilities, such as sarcopenia, frailty, inflammation, cognitive impairment, and dementia [10–13].
Food digestion begins in the mouth, with saliva secretion and mechanical mastication for the breakdown of food into small pieces. However, aging leads to a decrease in bite force by tooth loss and a reduction of oro-sensory receptors, resulting in a 50% decrease in saliva secretion and elevated taste thresholds/reduced sensitivity [14]. The second step is gastric emptying, which regulates the kinetics of nutrient absorption, and, in turn, nutrient utilization in body functions, as illustrated by the concept of slow/fast carbohydrates and proteins [15]. Pepsin and gastric acid secretion follow stimulation of the oral and gastric vagal afferents. The gastric emptying rate is dependent on the meal type (solid or liquid), other meal components, meal volume, caloric content, the types of dietary fiber, and the liquid-to-solid ratio of the meal. Some studies found that the halftime, indicating when half of the eaten meal is emptied, was 10-60 min for liquid meals but 50-115 min for solid foods [16,17]. In frail older adults, the gastric emptying time increased due to impairment of gastric motility, and gastric acid and pepsin were reduced by approximately 30% and 40%, respectively, due to chronic atrophic gastritis associated with Helicobacter pylori infection [18,19]. In the final stage of digestion, the digested meal is broken down into liquid in the small intestine, the main site of nutrient absorption. During digestion, the cells and bacteria lining the inner walls of the GIT break food down and absorb nutrients, while bile and pancreatic secretions assist digestion and absorption, and gut smooth muscles contract to move food through the GIT [15]. Although progress has been made in understanding how some of the components of the intestine are affected by aging, the comprehensive understanding is incomplete. However, a few studies found a significant increase in transit times in the aged colon and a reduction in the secretion of pancreatic enzymes (e.g., pancreatic lipase and chymotrypsin) with increased aging in animal and human models [20–22].
From the National Health and Nutrition Examination Survey (NHANES) 2003-2004, approximately one-third of American adults (> 70 years) insufficiently ingested the recommended dietary allowance for protein; moreover, approximately one-tenth of older women insufficiently ingested even the estimated average requirement of 0.66 g protein/kg/day [23]. The general recommended protein intake for older adults in the US and UK is 1.1-1.2 g/kg protein per day [24–27]. When comparing preferred foods and frequently consumed foods for 150 older adults in Korea, Kim and Lee found that although the most preferred food was meat (16.1%), the most frequently consumed food was soup/stew/steamed dish (16.0%), whereas the frequency of meat consumption was just 8.3% [28]. The meat was considered too difficult to consume due to tooth loss and a decline in mastication and digestive functions. In a study of the nutritional status of older adults, nutrient intake percentage and the component ratio of protein among energy intake rate from three major nutrients decreased with increasing deterioration of oral health status, suggesting that a reduction in mastication function affected protein intake [29].
Protein intake is especially important in older adults to overcome age-associated muscle anabolic resistance and to regenerate and maintain muscle mass as much as possible [30]. Meat contains essential amino acids and high levels of minerals (e.g., iron, zinc, and selenium) and B vitamins, and even a moderate intake can increase muscle protein synthesis in older adults [15], but meat texture is tough, fibrous, and difficult to chew. While meat proteins can be categorized as fast-digested proteins, this property depends on the masticatory efficiency. The decrease in masticatory efficiency of older adults can impair meat protein utilization for protein synthesis [31]. In order to improve the frequency of meat consumption in older adults, a strategy to improve meat protein digestion in older adults is needed that considers the age-associated decrease in masticatory ability/efficiency and digestive function. Such an approach would therefore involve the development of meat products with altered texture properties that are ideally suited for older adults. Since it is technically impossible to restore or control the effects of aging on physical function, the approaches need to increasing the digestibility of meat protein through pretreatment methods. Therefore, various pretreatment methods leading to changes in meat protein structure and digestibility that can be used in the meat industry and are targeted at older adults are presented in the subsequent sections of this review.
DIGESTION OF MEAT PROTEIN
Protein digestion mainly occurs in the stomach and small intestine, and proteins are absorbed as amino acids and small peptides in the small intestine. Gastric juice secreted in the stomach contains hydrochloric acid (HCl) and pepsin, a protease responsible for primary protein digestion. The highly acidic pH of the gastric fluid (approximately pH 2.0) has a potent antibacterial effect, rapidly killing microorganisms introduced into the stomach [32]. Pepsinogen (the inactive form of pepsin) is secreted from the stomach’s primary cells. It then reacts with the HCl secreted by parietal cells in the stomach and is converted to pepsin which, in turn, converts more pepsinogen into pepsin [33]. Pepsin requires an optimum temperature of 37°C, similar to body temperature, and an optimum pH of 1.8 (Fig. 1) [34]. The enzyme exhibits strong proteolytic activity, preferentially hydrolyzing peptide bonds involving the aromatic amino acids (tryptophan, tyrosine, and phenylalanine) at pH > 2.0 [35].
Protein and polypeptide digestion continues in the small intestine by the action of trypsin and chymotrypsin produced in the pancreas as trypsinogen and chymotrypsinogen, respectively, and secreted into the small intestine. Enteropeptidase converts trypsinogen to trypsin, which exhibits a particularly high affinity for peptide bonds after arginine or lysine, and trypsin activates chymotrypsinogen to chymotrypsin by hydrolyzing the peptide bond between amino acid residues 15 and 16 [36]. Chymotrypsin shows particularly high reactivity toward peptide bonds involving tyrosine, tryptophan, and phenylalanine and low reactivity toward peptide bonds involving leucine and methionine. The resulting tripeptides, dipeptides, and amino acids are absorbed through the blood vessels in the small intestine [37–39].
Protein intake and digestion rates directly affect human muscle synthesis, so it is important to improve these rates in older adults by facilitating protein digestion through various treatments of protein-rich foods [15]. When a large amount of protein is consumed, the secretion of digestive enzymes in the digestive system, intestinal peristalsis, and segmental movements increase. However, the reduced gastric acid secretion in older adults greatly decreases the action of pepsin. This, combined with the deterioration of intestinal muscles, reduces the rate of protein digestion and absorption [40]. Additionally, abdominal pain may increase, and various gastrointestinal diseases may flare up.
Methods for improving the digestion rate of meat are divided into chemical and physical methods (Fig. 2). Chemical methods include aging and adding, for example, calcium, sodium salt, phosphate, or a protease; dissolving a saline-soluble protein to increase digestion; or adding proteases derived from plants, microorganisms, or animals. Physical methods include sous vide, ultrasound, high pressure processing (HPP), and treatment using pulsed electric fields (PEF), which all destroy cells or tissues or alter the structure of meat proteins to increase their digestibility. For example, minced beef is more rapidly digested and absorbed than beef steak, resulting in increased amino acid availability and greater postprandial protein retention [41]. In addition, recent studies have reported an increase in the digestion of proteins by the action of microorganisms, such as probiotics, and an improvement in the digestion rate of proteins by controlling the gut microbiota [42,43].
The GIT is lined with mucosal epithelium, which acts as a natural barrier between the host and the luminal environment [44]. The intestinal barrier contains various components, including commensal gut microbiota, secretory immunoglobulin A molecules, antimicrobial peptides, mucus layers covering the intestinal epithelium, antimicrobial peptides, and junctional complexes (tight junctions, adherence junctions, and desmosomes).
On average, the number of bacteria in the duodenum and jejunum is 103–104 U/mL, increasing to 108 bacteria/mL in the ileum [44]. The critical contributions of gut bacteria toward human digestion have only been elucidated recently through primary degradation, amino acids (sulfur-containing-, basic-, and aromatic amino acids) degradation, pyruvate catabolism by the gut microbiome [45]. Many highly complex microorganisms exist in the GIT and play important roles in maintaining health and nutrient metabolism. The human GIT contains trillions of commensal bacteria [46]. Resident microorganisms in the human gut are influenced by factors such as birth, sex, health status, age, body weight, diet, physical activity, medicinal history, and usage of antibiotics [47]. The human gut microbiome plays a critical role in the digestion of the complex carbohydrates, protein components, and fats that reach the lower GIT by contributing enzymes not encoded by the human genome [45,48]. Five major bacterial phyla in the human digestive tract are Firmicutes, Bacteroidetes, Actinobacteria, Proteobacteria, and Verrucomicrobia. Firmicutes (Gram-positive) and Bacteroidetes (Gram-negative) make up the majority, accounting for approximately 65% of the total bacteria [37,49].
Approximately 25 g of protein enters the colon daily [50], and proteins are a major carbon and energy source for colonic bacteria. Although most dietary proteins are digested and absorbed in the small intestine, relatively high levels of residual proteins and peptides reach the colon and serve as substrates for fermentation by resident bacteria [51]. Some bacterial proteases degrade proteins to produce peptides and amino acids that can be fermented to generate short-chain fatty acids [37]. Bacteroides and Propionibacterium are the main proteolytic bacteria in fecal samples, but other common proteolytic bacteria include Clostridium, Streptococcus, Staphylococcus, and Bacillus [52]. Bacteria from the genera Clostridium, Fusobacterium, Bacteroides, Actinomyces, Propionibacterium, and Peptostreptococcus are involved in the in vitro proteolytic fermentation; bacteria from the genus Clostridium are important for processing lysine and proline through fermentation in the colon, whereas, Peptostreptococcus contributes to the catabolism of tryptophan and glutamate [53].
Probiotics function predominately in the large intestine [54]. Certain probiotic strains, such as lactic acid bacteria [55], can improve the protein digestibility of the host by increasing the activity of digestive enzymes [56]. Peng et al. reported that the core mechanism of probiotic action on protein metabolism is the remodeling of the host intestinal microbiota because microorganisms directly participate in the metabolic process of dietary proteins [57]. As described in the review by Wang and Ji, the probiotic Bacillus coagulans GBI-30 increased the digestion and uptake of three nutritious plant proteins in the upper GIT, and the oral administration of Lactobacillus plantarum GF103 and Bacillus subtilis B27 to Holstein calves improved the apparent digestibility of crude protein over 8 weeks [46]. Hu et al. reported that Bacillus amyloliquefaciens significantly enhanced chymotrypsin activity in the jejunum and ileum [58].
Probiotics modulate intestinal microbiota through colonization and exclusion of pathogens [57]. Moreover, probiotics can alter the intestinal microbial environment and enhance intestinal immunity, increasing resistance to diseases, reducing pathogenic infections and disease symptoms, and improving health [48]. Piglets consuming Lactobacillus strains expressed 32, 40, and 27 proteins that maintain the integrity of cell structures, pathogen defense, and cell stability, respectively [59]. Yi et al. reported that probiotic Lactobacillus reuteri LR1 was associated with increases in both villus height-to-crypt depth ratio and tight junction protein expression in the mucosa of the jejunum and ileum [60]. Storelli et al. reported that L. plantarum activates cell growth signaling pathways in gut enterocytes, increasing protein metabolism in the gut [61]. Kimmel et al. reported that B. coagulans GBI-30, 6086 improves the health of cells of the gut lining by improving nutrient absorption, reducing inflammation, and inducing optimum development of the absorptive area in the villi [62]. This same probiotic strain can increase protein absorption under in vitro conditions [63]. Toohey et al. revealed that B. subtilis supplementation might improve body composition by enhancing the absorption and utilization of dietary protein, thereby increasing dietary protein-induced thermogenesis and changing satiety signals [64]. The metabolism of peptides and amino acids by gut bacteria can result in a wide range of metabolites, including nitrosamines, heterocyclic amines, and hydrogen sulfide, some of which are harmful and genotoxic and have been linked to colon diseases [65]. Probiotics improve the functioning of the digestive system by enhancing the function of the small intestine wall (villus) and suppressing harmful bacteria. They are thought to have a positive indirect effect on food digestion rate.
Chemical methods to hydrolyze protein bonds or fragment myofibrils and muscle fibers involve adding factors that affect enzyme activation or adding the enzyme itself. Calpain is a calcium-activated protease and is generally present in the muscle tissue, and its activity is the most important reaction in the aging of meat. Calcium ions in the muscle tissue of livestock are released and react with calpain, resulting in a proteolytic reaction within the muscles. Thus, the addition of calcium ions can increase the activity of calpain and, thereby, proteolysis [66]. Conversely, adding sodium and phosphate to meat using this principle destroys the existing structure of actin and myosin to form a gel, thus increasing the digestibility of the proteins [67]. Actin and myosin, which account for at least 50% of meat protein, are saline-soluble proteins that are soluble when the ionic strength is about 0.3 M or more [68].
Three plant digestive enzymes (i.e., papain, bromelain, and ficin), malt, and the microorganisms B. subtilis var. amyloliquefaciens, Aspergillus niger, and Rhizopus oryzae are recognized by the FDA as Generally Recognized as Safe (GRAS) [69]. Proteases are widely used in food and milk processing and pharmaceutical and medical industries. However, not all proteins are applicable for food applications. Recombinant proteases produced by genetically engineered microorganisms are not available in some countries, and animal-based proteases are difficult to use in the food industry because of the risk of zoonosis [70]. By contrast, plant proteases have a long history of being used as food or additives, such as for improving the tenderness of meat products [71], and were registered GRAS by the FDA in 1997. Moreover, their extraction is simple and low-cost, and their preparations have no pathogenic potential for humans or animals. Oral toxicity experiments in mice show that plant proteases have very low toxicity, with an median lethal dose (LD50) above 10 g/kg [72].
Plant proteases, also known as cysteine and thiol proteases, include papain, bromelain, ficin, actinidin, and zingibain. Cysteine proteases commonly have an imidazole ring situated near the cysteine residues. The imidazole ring in cysteine proteases reacts with the amino acids, causing a deprotonation reaction. Afterward, the cysteine in the enzyme causes hydrolysis of peptide bonds through nucleophilic substitution with the carbon of the carbonyl group of amino acids. This reaction occurs throughout the protein, not only at their ends. Cysteine proteases have low substrate specificity, enabling the hydrolysis of various binding sites, such as amide bonds, ester bonds, and thiol ester bonds [73]. In a recent study, when actinidin was added to a beef brisket at a level of 10% (w/w) and cooked at 70°C, there was no significant difference in pH, color, or cooking loss compared with the sample without the enzyme. Sensory evaluation showed higher sensory scores for tenderness, juiciness, and flavor, and sodium dodecyl sulfate-polyacrylamide gel electrophoresis (SDS-PAGE) showed increased levels of proteolysis in the samples with the enzyme [74].
Papain or papaya protease I is extracted from papaya latex, and its proteolytic properties have long been known due to its use as a meat tenderizer on proteolytic effects [75]. Papain has a molecular weight of 23.4 kDa and comprises 212 amino acids. The proteolytic mechanism of papain is manifested by the reaction of an imidazole ring linked to His159 by Asp175, which causes a deprotonation reaction and hydrolysis by Cys25 [76]. Papain shows proteolytic ability between pH 3.0 to 12.0, with an optimum temperature of 65°C, which is much higher than those of most enzymes, and an optimum pH for activity of 6.5–7.5 [77,78]. In an experiment where meat was treated with proteases, such as papain, the myofibrillar protein, a major muscle protein, was metabolized, and the binding force of connective tissues, such as collagen, which is generally poorly digested, was impaired [66].
The proteolysis reaction of papain starts with a nucleophilic substitution, in which the thiol group of Cys25 reacts with a carbonyl group of proteins. Through this reaction, the thiol group of papain forms a tetrahedral intermediate separated from Cys25. Intermediate metabolites are highly unstable and quickly react with the hydrogen in the imidazole ring and collapse. The collapsed metabolite regenerates the carboxyl group of Cys25 to form amine R-NH2. Subsequently, the carboxyl group reacts with a water molecule and regenerates the thiol group of Cys25 and the imidazole ring to terminate the proteolysis reaction [79,80]. Papain can hydrolyze the bonds between arginine and non-valine amino acids, followed by those between hydrophobic amino acids, such as alanine, valine, and leucine [81] (Fig. 3A).
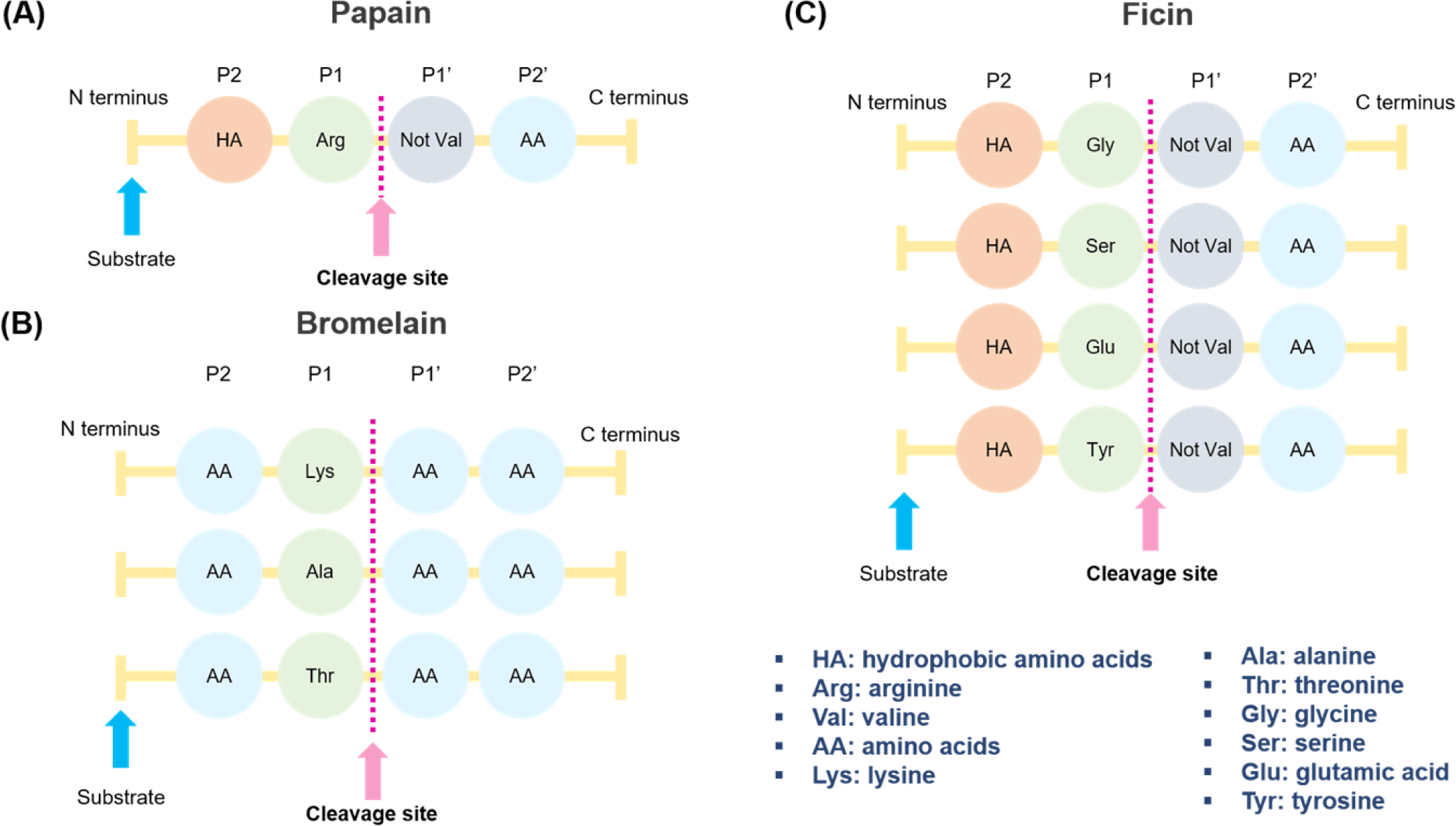
During papain treatment on beef, the free amino acids concentration and the meat tenderness increased with the treatment time and concentration [71]. In addition, tenderness increased when papain was added to beef and chicken patties [82]. Experiments showing the proteolytic effects of papain and bromelain on pork through SDS-PAGE analysis demonstrated that the experimental group treated with papain had a lower protein molecular weight than the bromelain treatment group [83]. However, in other similar experiments with beef, the SDS-PAGE results did not show significant differences after papain and bromelain enzymatic action [84]. This inconsistency could be due to differences in the protease cleavage site or the detailed experimental methods. Ionescu et al. reported that papain activity on the polypeptides of beef was higher than that of bromelain, increasing the content of free amino acids [85]. When comparing papain with other cysteine proteases, papain had a greater effect on the connective tissue in meat, such as collagen, while the other enzymes mainly affected myofibrillar protein [86]. Papain hydrolyzes the heavy chain of muscle myosin (approximately 94 kDa) from the N-terminus to give subfragment S1 head and tail [87].
Bromelain is a cysteine protease in pineapple (Ananas comosus), mainly in the stems and pulp. Pulp bromelain is a functional group of enzymes bound to aspartic acid but not cysteine. Stem bromelain has a reduced proteolytic capacity and a lower specificity for peptide bonds than pulp bromelain [70,77]. Bromelain used for industrial applications generally has low substrate specificity, enabling the peptide to be metabolized into several fragments. Stem bromelain is mainly used because of its economic feasibility, as it can be purified from the stems. Stem bromelain consists of 285 amino acids, has a molecular weight of 33 kDa and contains seven cysteines. The functional pH range is 6.0-7.0, and the optimum temperature is approximately 50°C [77]. Bromelain can hydrolyze the peptide bonds of amino acids combined with lysine, alanine, and threonine (Fig. 3B). Unlike papain and ficin, bromelain can be broken down by any amino acid (AA) at the P2 and P1’ sites; thus, it can release to a very wide range of areas in the protein.
When bromelain was added to the sous vide cooking process, there was a significant softening effect on meat quality and an increase in storage period, whereas there was no observed increase in the digestion rate [88]. In an experiment comparing the collagen breakdown capabilities of several cysteine proteases, actinidin and bromelain were found to be particularly effective in collagen decomposition and are thus expected to have a high connective tissue breakdown effect when applied to meat [88]. An experiment comparing the ability of bromelain to hydrolyze myofibrillar protein showed a significant breakdown effect compared to other cysteine proteases [84]. In the treatment of beef, bromelain showed better proteolytic effects and increased the free amino acid content of the meat compared to papain [85]. The relationship between proteolysis during tenderization by bromelain and meat protein digestibility in beef was evaluated using an in vitro simulated digestion model [89]. After tenderization with bromelain, microstructure disruptions were observed, such as around the Z-discs in meat. Furthermore, the addition of bromelain exhibited higher the degree of hydrolysis than in vitro digestion without bromelain. These results proved that the use of bromelain affected tenderization or digestibility of meat protein [89].
Ficin is a protease extracted from fig latex and is widely used in the food industry as an enzyme for softening meat [77]. The optimum pH range of ficin is 6.5–9.5, showing high activity over a relatively wide range, and the optimum activity temperature is 45°C–55°C. It consists of a single polypeptide chain with a molecular weight of approximately 26 kDa [90]. Although the substrate specificity is low and the proteolytic ability is excellent, it shows long-term instability; the activity is reduced by half after 90 min at 60°C [91]. Ficin can hydrolyze peptide bonds with glycine, serine, glutamine, and amino acids following tyrosine linked to hydrophobic amino acids (Fig. 3C). Compared to other enzyme solutions (papain, bromelain, Aspergillus oryzae concentrate protease, Aspergillus oryzae 400 protease, Bacillus subtilis protease, and ginger), ficin is the effective enzyme in meat such as Triceps brachii and Supraspinatus, resulting in a higher level of water-soluble proteins at 69.3 ± 0.25 mg/g of meat [92]. Kaur et al. reported that enzymes acted randomly and uniformly on raw meat myofibrils [87]. By contrast, the enzyme action started from the edges of cooked meat myofibrils and moved toward the center as digestion progressed.
There have been many studies to improve meat tenderness using plant-derived proteases [77,88]. Beyond their use for meat tenderization, plant proteases have been shown to increase meat protein digestibility due to protein breakdown associated with ultrastructural changes during simulated digestion in vitro [74,93]. Although plant-derived protein enzymes have proteolytic effects, such as collagen decomposition and myofibrillar protein breakdown, which lead to improved meat tenderness, further studies are needed to prove the relationship between the application of plant-derived proteases and changes in the digestibility of meat proteins.
Physical methods to improve protein digestion can be divided into methods causing protein degeneration through heating or destroying the muscle tissues and cells by applying physical force directly. Protein structure changes with increased heating temperature and time. The tertiary structure of proteins changes when heated at 50°C–60°C or higher, and the secondary and tertiary structures denature when the protein is heated at 60°C–90°C for more than an hour [94]. When a protein is denatured, the bonds maintaining the protein structure are weakened, and the non-polar area inside the protein structure is exposed, increasing the surface area and hydrophobicity of the protein and resulting in an increase in the protein digestion rate [95]. However, prolonged heating of proteins at temperatures above 100°C can cause extensive myosin aggregation in meat, which can interfere with enzyme-mediated proteolysis [96]. Kaur et al. reported that cooking conditions affected in vitro protein digestion, but extended cooking at 100°C did not increase digestibility [87]. Wen et al. found that protein digestibility decreased with an increase in core temperature, which could be attributed to protein aggregation [97]. Due to the low temperature of approximately 60°C–80°C under a vacuum, the sous vide cooking method can suppress protein aggregation, and the digestion rate can be increased by increasing the total surface area of the protein [98,99]. In an experiment measuring the digestion rate of pork according to the actual cooking temperature, pork heated at a temperature above 100°C showed a slower digestion rate than pork cooked at a low temperature of 70°C–80°C. It also showed lower susceptibility to exogenus proteases [100]. Bax et al. reported that protein digestion could be regulated by meat preparation, with slower digestion observed at higher cooking temperatures [101]. Yin et al. reported that sous vide significantly accelerated the release of cathepsin B and cathepsin L from lysosomes, increased the breakdown of the myosin heavy chain, increased the collagen solubility and myofibrillar fragmentation, and resulted in a longer sarcomere length compared to control samples cooked at 75°C [102]. Liu et al. reported that with increasing temperature (50°C, 60°C, and 70°C) and time (15 and 30 min), the digestibility of sturgeon myofibrillar protein decreased, whereas the particle size and protein aggregation increased [103]. However, sous vide cooking with low-temperatures (50°C, 60°C and 70°C) relieved the heat stress of myofibrillar protein conformation and reduced protein aggregation, which positively influenced the enzymatic hydrolysis of myofibrillar proteins, thus improving the digestibility of sturgeon myofibrillar proteins [103]. Regarding the secondary structure of the myofibrillar protein, the content of the α-helix in the low-temperature vacuum heating group was reduced from 17.25% to 11.99% with increasing temperature and time, whereas the change in the content of the β-sheet increased from 32.96% to 42.13% with increasing temperature and time and then decreased [103].
Kehlet et al. reported that cooking at 70°C increases protein digestibility due to denaturation increasing the approachability of cleavage sites to gastrointestinal enzymes compared to 100°C or above [104]. However, cooking at high temperatures or for a prolonged time can induce protein-protein interactions, leading to aggregation [103,104]. Protein aggregation limits the accessibility of enzymes during digestion and thus may slow the digestibility of oven-cooked pork [104]. Kehlet et al. concluded that the gastric digestion of meat proteins in vitro was faster after 72 min at 58°C compared to oven cooking at 160°C and a longer low-temperature holding time of 17 h [104]. The general temperature and time recommended by chefs for sous vide cooking beef, pork, and lamb range from 58°C to 63°C for 10–48 h [105,106]. Numerous connective tissues in muscles require longer sous vide times than tender meat cuts. Baldwin reported that cooking at temperatures between 55°C and 60°C for 24–48 h was suitable for softening tough meat cuts (pork shoulders and beef chuck) [105,107]. Summarizing the mechanisms for increasing protein digestion by thermal treatments, it was found that thermal treatments destroyed the primary and secondary structures of the protein, and the digestion rate of the protein was increased due to α-helix reduction and β-sheet increase (Fig. 4).
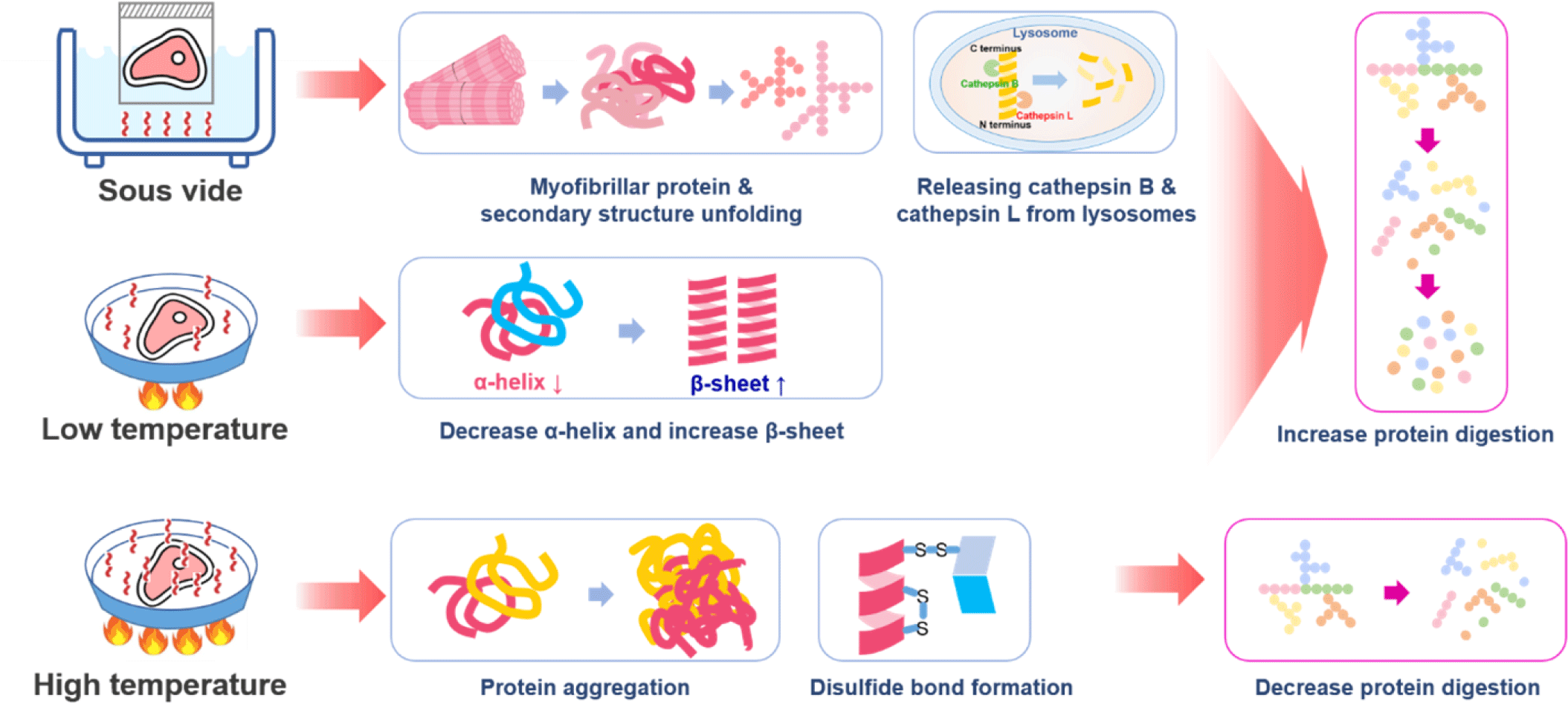
Ultrasound, HPP, and PEF apply a physical force directly to a protein. Ultrasound is a green food processing technology. High frequency and low field strength (100 kHz−1 MHz, < 1 W/cm2) are widely used for the non-destructive testing of food and to inhibit microorganisms and enzymes for preserving food quality, while low frequency and high field strength (20–100 kHz, > 1 W/cm2) is used to alter protein molecules [108]. The application of ultrasound to liquid systems causes acoustic cavitation, which is the phenomenon of the generation, growth, and eventual collapse of bubbles [109]. As ultrasound waves propagate, the bubbles collapse and oscillate with mechanical (turbulence or shear stress) and chemical effects [109]. Ultrasound causes the hydrolysis of water inside the oscillating bubbles, which induces the formation of H+ and •OH free radicals; free radicals can be scavenged by amino acids of the enzymes involved in substrate binding, structural stability, or catalytic functions [109].
Ultrasound (20 kHz) offers a physical method to increase meat tenderness and digestion rate. When ultrasonic waves are applied to meat, a vacuum space is created in the medium, such as water, owing to cavitation, and the generated energy transmits a very high shear force to the meat. Non-covalent bonds between proteins produced by the cavitation effect and mechanical oscillation may be destroyed by the turbulence and microcurrent induced by ultrasound, which leads to structural and functional alteration [110]. As a result, tissues and cells in the meat are destroyed, increasing the tenderness of the meat and interfering with chemical bonds that determine the shape and function of the protein by destroying and unfolding the protein structure [111,112].
After ultrasound application to meat, observations confirmed that a gap was formed between the muscle fibers and that the sarcomere structure was destroyed [113]. Ultrasound treatment of semitendinosus muscles from beef increased the protein digestion rate of beef, and the SDS-PAGE results showed a decreased content of high molecular weight protein and increased content of low molecular weight protein [114].
Ultrasound treatment can enhance the solubility of myofibrillar proteins by increasing the pH and reducing the protein particle size [110]. Solubility is a prerequisite for other functional properties, such as water-holding capacity, emulsifying properties, and foaming properties, and gel strength was improved considerably after sonication [110]. Many proteins are functional in their soluble form, and protein solubility is the most practical indicator for protein denaturation and aggregation. Myofibrillar protein solubility increased with ultrasound power and treatment time [110]. From these results, the increase in protein solubility seems to be associated with the reduction in myofibrillar protein size and enhancement of protein-water interactions due to an increase in surface area after ultrasound treatment [110,115,116].
Protein digestibility depends on the local flexibility of the substrate molecule [117]. This determines the quantity of exposed and applied cleavage sites for hydrolysis and how easily cleavage sites on the protein can be bound with digestive enzymes [117]. Ultrasound can promote protein hydrolysis by inducing alterations in protein structure, resulting in the exposure of enzyme cleavage sites and thereby increased protein digestibility [117]. Bagarinao et al. reported that raw ultrasound-treated samples (in water or enzyme solution) showed degradation of the muscle fibers and exhibited an expansion of the extracellular spaces [118]. Ultrasound-treated cooked samples had large spaces between myofibrils, which were less obvious in samples ultrasonicated in an enzyme solution [118]. The main method to improve protein digestion with ultrasound treatment is to soak the meat in water and apply ultrasound, which can cause cavitation effects leading to muscle myofilament collapse, a reduction in the myofibrillar protein size, and hydrolysis of the meat protein (Fig. 5).
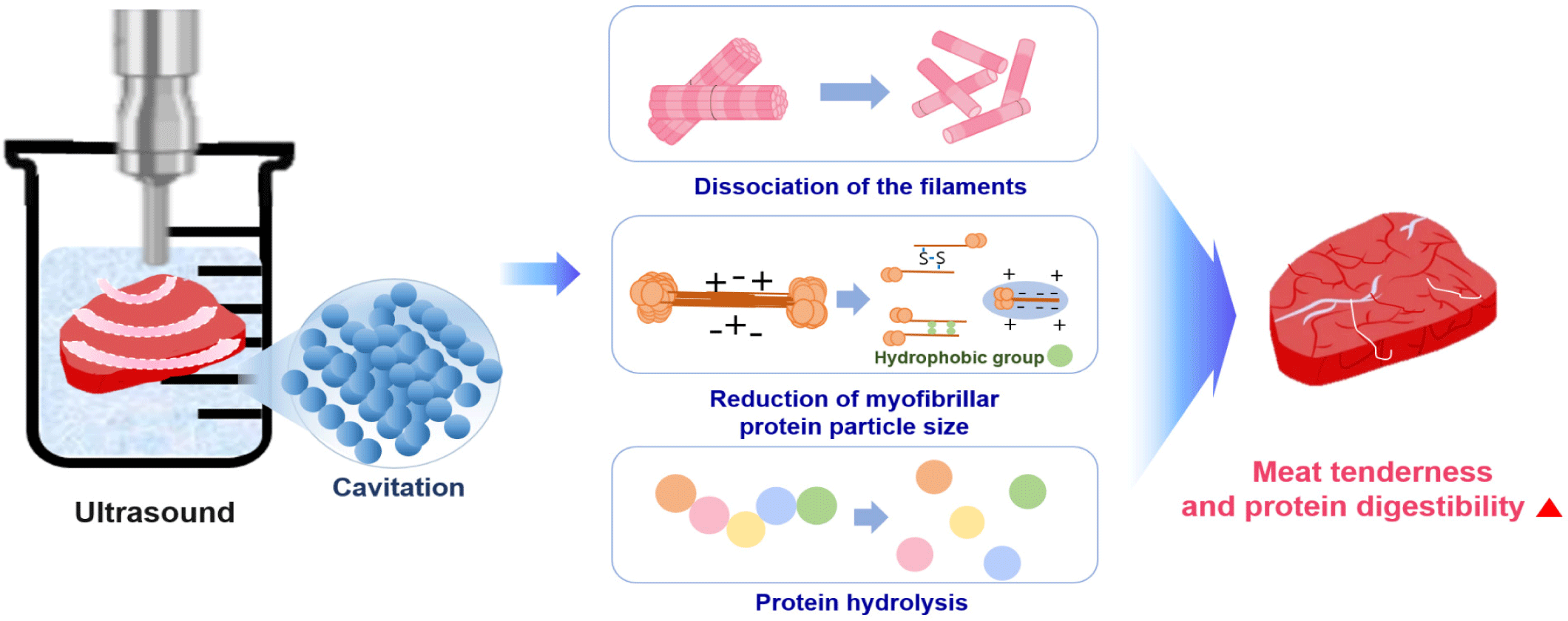
HPP is a food preservation technique without thermogenesis that prohibits harmful pathogens and vegetative spoilage microorganisms by using pressure rather than heat. HPP uses intense pressure (approximately 400–600 MPa or 58,000–87,000 psi) at chilled or mild processing temperatures (< 45°C), allowing most foods to be preserved with minimal impacts on nutritional value, appearance, taste, and texture [119,120].
The working principle of HPP is as follows: hermetically sealed food products are placed in a thermally insulated airtight container and receive ultra-high pressure (100–600 MPa) transferred by a liquid medium (commonly water), which provides a pasteurization effect via the application of high pressure. According to the principle of compression heating, an increase of approximately 3°C in the water temperature occurs with an increase in pressure of 100 MPa [121].
Cao et al. reported that HPP affected the secondary, tertiary, and quaternary protein structures to different extents. In particular, high pressures (> 700 MPa) can cause irreversible denaturation by interrupting the secondary structure of proteins [122]. At > 200 MPa, the tertiary structure was changed due to the alteration of the hydrophobic and disulfide bonds, whereas quaternary structures were affected by pressures in the range of 100–150 MPa [122]. These changes in protein structure have profound effects on the functionality of a protein and its possible food applications [122].
Owing to its advantage of sterilizing microorganisms without heating, HPP has been since the early 2000s on fresh foods that are difficult to heat-treat [123]. Since then, HPP has been shown to increase the tenderness of the meat and the digestion rate of protein, and it is gradually being used in various home meal replacement (HMR) products, such as sausages and gels. HPP is reported to increase the digestion rate of meat by causing protein denaturation and tissue cell damage. However, the overall quality reduction is less than the heat-treatment method because it does not significantly affect amino acids, flavoring ingredients, or vitamins [123,124]. HPP has been reported to involve protein denaturation, degradation, or gelation, depending on the protein system, temperature, and the pressure treatment condition (time and pressure level) [124,125]. Protein denaturation occurs during HPP due to the destabilization of non-covalent interactions in the tertiary structure, particularly hydrophobic and ionic interactions [124,126]. The HPP-induced changes begin with the fragmentation of myofibrils [127]. The initial step is I-, M-, and Z-line disruption when the pressure level reaches 200 MPa, resulting in the breakdown of the myofibrillar structure [127]. High pressure induces myofibrillar protein solubilization by causing the dissociation of the thin and thick filaments to liberate soluble components from myofibrils [127]. HPP technology has been developed as a non-thermal pasteurization technology in the meat industry to improve microbiological safety and shelf life. HPP leads to increased permeability and leakage of meat cell contents, such as protein hydrolysis enzymes, ultimately resulting in accelerated digestion of meat protein. Rakotondramavo et al. reported that HPP decreased the digestibility of cooked ham because the denaturation and oxidation phenomena leading to protein aggregation masked the cleavage sites required by the digestive enzymes [128]. Therefore, each step of the high-pressure cooked ham processing impacted the protein digestion parameters: the curing step enhanced the digestibility and proteolysis rate of protein, whereas the cooking and high-pressure treatments reduced the digestibility and proteolysis rate of pork protein [128].
Post-mortem changes in the muscle depend on the endogenous protease activity [129]. Calpain and other proteolytic enzymes decompose myofibrillar proteins, including Z-line proteins, causing myofibril fragmentation [129]. Ohmori et al. reported that HPP at 303.975–506.625 MPa denatured tissue proteins and increased their proteolytic susceptibility [129]. They summarized that applying high pressures of 101.325–202.65 MPa to meat may enhance the endogenous proteolytic activity participating in meat conditioning by releasing proteases from lysosomes and denaturing the tissue protein. Chun et al. revealed the enhanced hydrolyzing activities of three selected proteases (pepsin, trypsin, and chymotrypsin) induced by HPP at around 200 MPa [130]. Trypsin showed the best collagen-hydrolyzing activity. Pressurization at 100-200 MPa was responsible for improving proteolytic activity, although it was unclear whether an interaction between the enzyme and substrate occurred under pressure or whether structural modification of the enzymes caused the enhancement of the hydrolysis reaction [130]. HPP can induce the protein unfolding and extension of peptides exposed to some internal groups, including hydrophobic groups and inter-sulfhydryl groups. Therefore, HPP treatment affected the hydrolysis, and the HPP-treated products showed high digestibility with high percentages of low molecular weight proteins and peptides (< 1 kDa) [42]. Franck et al. reported that an increase in the abundance of smaller peptides (500–1500 Da) at higher pressures corresponds to an increase in the degree of hydrolysis [131]. This may be related to two reactions: high-pressure-induced enzyme activation or high-pressure-induced protein unfolding [131]. Some study have suggested that pressure-induced protein unfolding facilitates access to trypsin cleavage sites (or C-terminal bonds of lysine and arginine), increasing enzyme activity and hydrolysis [131]. This is because hydrolysis increases with increasing pressure and pressurization time. High pressure has been reported to cause protein denaturation and gelation, the collapse of filaments, and the depolarization of myofibrils in meat (Fig. 6).
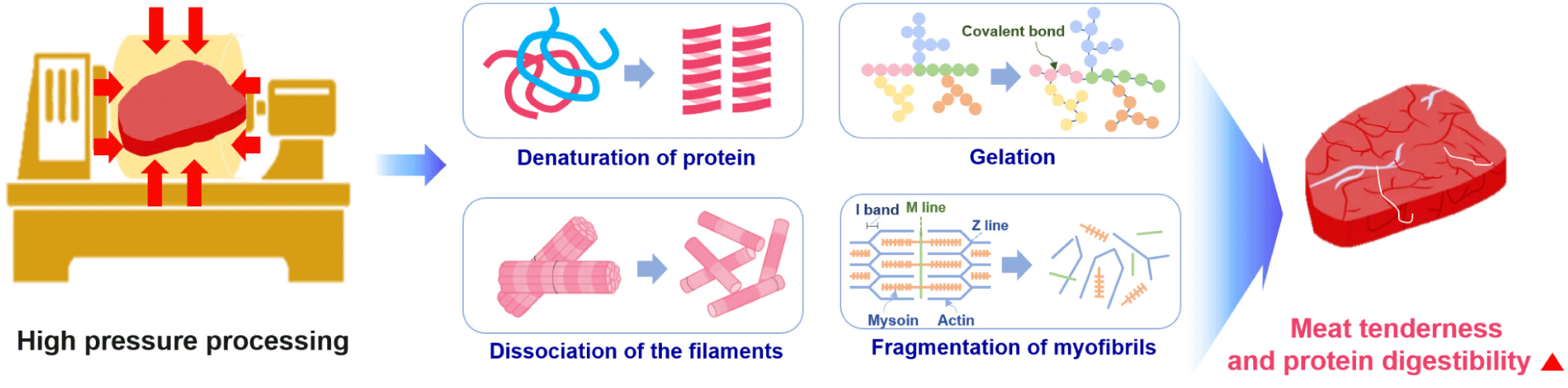
PEF processing involves the application of high-voltage pulses for short durations to food placed between two electrodes [132]. The PEF equipment includes a pulse generator, a chamber, electrodes designed to avoid the impact of electrolysis, a control system, and a data acquisition system [133]. There is a field threshold value of approximately 1–10 kV/cm depending on the sample type (e.g., plant, microbial, animal). When that is exceeded, the electrocompressive force induces a local dielectric breakdown of the cell membrane, creating a pore that can function as a conductive channel [132]. When PEF disrupts the cell membrane, intracellular contents leak out, resulting in the loss of cell metabolic activities [134].
Recently, PEF treatment was reported to increase the digestion rate of proteins [135,136]. The electric field is posited to ionize various substances inside the meat and cause chemical reactions, such as altering the secondary and tertiary structures of meat proteins [137]. The mechanism of the changes in protein structure caused by PEF has not yet been accurately defined. However, related studies have shown that protein molecules are polarized at a low PEF strength, and their hydrophobic amino acids gradually become exposed to the solvent as the electric field strength increases. At a relatively high field strength, aggregation of the unfolding proteins may occur through weakly covalent and non-covalent bonds [138]. Above certain PEF strengths, the thermogenesis produced by arcing would play a crucial role in the denaturation and aggregation of heat-sensitive proteins [138]. Zhao and Yang demonstrated that PEF could increase the extrinsic fluorescence intensity in lysozyme through the presence of more hydrophobic groups being exposed to solvents [139]. The content of β-sheets and unordered structures also increased along with a reduction in the α-helix. Therefore, PEF can simultaneously damage the secondary and tertiary structures of lysozyme [138].
Physical treatment methods, such as PEF, destroy muscle tissue to create space between the cells, and they can increase the effectiveness of proteolytic enzyme treatment, affect the cells that form the muscle tissue, weaken the function of sarcoplasm, and destroy lysosomes to release calcium ions and calpain. Calpain, a proteolytic enzyme in cells, is activated by contact with calcium outside the cell, promoting the autolysis of meat and increasing the protein digestion rate [140]. When applied to beef, PEF treatment increased the rate of in vitro digestion by approximately 20% due to the weakening of the binding force of muscle tissue without affecting the color and pH [136]. Similarly, the protein digestion of deer meat was increased by PEF treatment, as confirmed by SDS-PAGE analysis [137,139]. These results suggest that PEF-induced electroporation might have enhanced the effect by facilitating the penetration of digestive enzymes into the muscle matrix [139]. They also demonstrate the potential commercial viability of PEF for enhancing the protein digestibility of meat [135].
In addition to the positive effects of PEF treatment on protein breakdown and protein digestion rate, PEF-induced electroporation of the cell membrane accelerates the release of calcium ions and μ-calpain, promoting glycolytic processes for early proteolysis, which improves meat tenderness [73,141]. However, PEF can also tenderize meat through other mechanisms besides electroporation, such as the degradation of muscle fiber structure and breakdown of myofibrils through the Z-line of muscle fibers [105,142]. According to Zou et al., fiber type could be the key factor in explaining the differences in protein susceptibility to digestion [112]. The effect of an electric field on the binding of proteins and peptides in meat remains elusive, but it is surmised that the main mechanisms for the increased tenderness and protein digestibility of meat by PEF are protein denaturation, muscle fiber depolarization, and myofibril destruction (mainly Z-line) (Fig. 7).
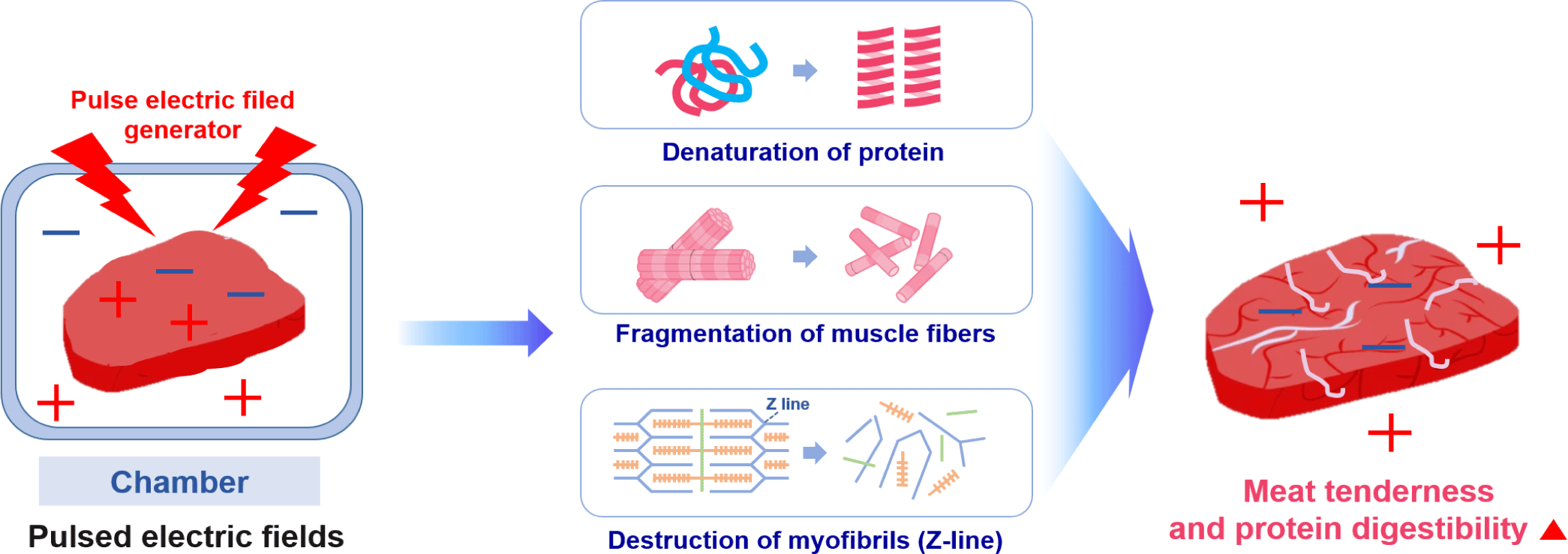
Currently, non-thermal treatment methods, such as ultrasound, HPP, and PEF, are mainly used for vegetables and fish, which are easily degraded during thermal treatment, although research suggests that such methods are also sufficiently effective for treating meat [135,137]. In this review, the impact of improving the digestion rate of meat proteins by physical treatments, such as thermal, ultrasound, HPP, or PEF, is thought to be similar. Presumably, this is because the structure of the muscle fibers is destroyed and fragmented, the chemical bonds are weakened, and the proteins are reduced in size or hydrolyzed by physical treatments, thereby increasing the contact area between the protein and the digestive enzyme and the efficiency of the digestive enzyme. Since improving protein digestion through physical methods is thought to have a relatively small effect on the flavor or taste of meat products compared to plant-based protein digestive enzymes, it is necessary to select the optimal method considering the sensory characteristics of meat products when developing products.
CONCLUSION
Various methods are available to increase the digestibility of proteins, with implications for increasing the consumption of protein-rich foods, especially meat, thereby improving protein utilization for older adults. These methods are gut microbiota and probiotics; chemical methods, including aging and enzymatic treatment (plant-derived proteases); and physical methods, including heat, ultrasound, HPP, and PEF. There is substantial evidence emerging to suggest that diet composition plays an important role in shaping the gut microbiome and that various diet components may impact the gut microbiota composition. In this context, the digestibility of proteins may depend on the gut microbiota. However, further research is necessary because studies regarding the relationship between gut microbiota and protein digestion are still insufficient. Probiotics can improve the digestion of proteins by improving the function of the GIT and secreting enzymes.
Plant proteases are the most focused research area for increasing the digestibility of proteins by chemical methods. The chemical and physical methods disrupt the structural integrity of meat protein and dissociate connective tissues, muscle fiber, and myofibrils, with potential implications for improving meat protein digestion in older adults. However, clinical trials on products with improved protein digestion for older adults are currently insufficient, as are studies on the effect of chemical or physical treatment on the sensory properties of foods. Therefore, studies, such as clinical trials and sensory evaluation, of products treated using methods to improve protein digestion in older adults should be conducted.