Background
Mesenchymal stem cells (MSCs) are one of the best candidates for cell therapy and regenerative medicine because of their self-renewal, multilineage differentiation, immunomodulatory capabilities and limited tendency to tumorogenesis [1,2]. Many studies have been reported the application of MSCs for treatment of equine musculoskeletal disorders [3,4]. Equine MSCs could be isolated from different sources, including bone marrow, adipose tissue, and umbilical cord [5,6]. Among the sources of equine MSCs that have been isolated so far, bone marrow and adipose tissue are the main sources in clinical trials for treating equine orthopedic disorders [7]. Although MSCs- derived from various sources have similarities, some differences have been reported in biological, proliferative, immunological and differentiative characteristics [8]. MSCs are different in gene expression profile [9,10] and microarray analyses of stemness markers in equine MSCs have shown differences in molecular phenotypes [11]. These differences lead to different functional potentials based on anatomical location [12]. Different characteristics of MSCs can be detectable at transcriptional or translational levels. As a limited specific monolclonal antibodies are available for phenotyping of equine MSCs using immunostaining and flowcytometry techniques, comparative gene expression analysis on mRNA level using quantitative real time- polymerase chain reaction (qRT-PCR) has a great value [13,14].
qRT-PCR is a popular mean to evaluate mRNA expression level and a sensitive and accurate technique for amplification of mRNA [15]. In this method, amplicon accumulation is measured by intercalating dyes such as SYBR Green I [16,17]. However, it is essential to control for errors between samples when measuring RNA expression. Therefore, coincident measurement of a reference gene- also called housekeeping gene- should be used for the normalization of target gene expression data. There are some various reference genes which are involved in different processes in the cells [18].
In the relative quantitative RT-PCR method, an appropriate reference gene should be considered for accurate quantification of RNA expression because the cycle thresholds (CT) of the target genes are compared to the reference gene(s) [19]. The expression levels of reference genes should be remained constant between the cells isolated from different tissues [20], otherwise the normalization to varying internal references can result to increased errors [16]. Several genes have been used as housekeeping genes, including β2-microglobulin (B2M), glyceraldehyde 3-phosphate dehydrogenase (GAPDH), β-actin (ACTB), hypoxanthine phosphoribosyl transferase (HPRT) and ribosomal RNA (18 s and 28 s rRNA) [18,21]. From these genes, β-actin, GAPDH and B2M are used most frequent as reference genes in comparative gene analysis in researches on equine marrow- and adipose- derived MSCs [22-24]. GAPDH catalyzes the oxidative phosphorylation of glyceraldehyde 3-phosphate to 1,3-bisphosphoglycerate during glycolysis as well as the reverse reaction in gluconeogenesis. β-actin is one of the cytoskeletal actins that are involved in cell motility, structure and integrity [18,25]. B2M is a structural protein and a component of MHC-I molecule. B2M has positive or negative effect on cell proliferation depending on cell type [26,27]. It seems that cell source and experimental treatment can affect the expression level of housekeeping genes [28]. Therefore, it is important to evaluate the stability of housekeeping genes expression between MSCs- derived from different sources.
The aim of this study was to investigate whether the source of mesenchymal stem cells (MSCs) has any effect on expression level of three common reference genes (GAPDH, β-actin and B2M) in equine marrow- and adipose- derived undifferentiated MSCs and consequently their reliability for comparative qRT-PCR.
Methods
The experimental protocols were approved by the Committee of Ethics and Animal Welfare of the School of Veterinary Medicine and Animal Science, Ferdowsi university of Mashhad, Iran. Bone marrow (BM) and adipose tissue (AT) samples were collected from three healthy mares aged 3, 6 and 10 years old. BM samples were aspirated using a Jamshidi needle with 1000 IU of sodium heparin/ml. Mononuclear cells were isolated by gradient centrifugation on Histopaque®-1077 (Sigma-Aldrich) for 30 min at 400 g. The cells were rinsed twice with Dulbecco’s phosphate buffered saline (DPBS−, Gibco) and plated at 8 × 105 cells/cm2 in 25 cm2 flasks in growth medium consisting of high glucose Dulbecco’s Modified Eagle’s Medium (DMEM, Sigma-Aldrich) supplemented with 10% Fetal Bovine Serum (FBS), and 1% Streptomycin/Penicillin and 0.1% amphotericin.
Samples of adipose tissue were collected from gluteal region. The stromal vascular fraction (SVF) was immediately isolated by digestion with 0.1% of collagenase (Type I, Sigma–Aldrich) supplemented with 1% Bovine Serum Albumin (BSA), in an incubator at 37°C for 2 hours. The final solution was centrifuged at 600 g for 5 minutes and the cell-containing pellet was resuspended. The cells were washed twice with DPBS− and seeded at 8 × 104 cells/cm2 in 25 cm2 flasks in growth medium similar to bone marrow- derived cells.
Cells were incubated at 37°C at 5% CO2 until reaching approximately 80% confluence. The cells were then treated with Tryple enzyme (Invitrogen) and passaged until 3rd passage (P3). P3 cells (3 × 106) were transported to microtubes and stored at −80°C for total RNA extraction.
To characterize isolated cells at P3, they were examined for triliniage differentiation capacity (osteogenic, chondrogenic and adipogenic) using defined media. In addition, expression of specific cell surface markers including CD29, CD90, CD34 and MHC-II were investigated.
Frozen cells (stored at −80°C) were used for RNA extraction. Total RNA of each sample (containing 2 million cells) was extracted with high pure column RNA extraction kit according to manufacturer’s instructions (Roche, Germany). The RNA concentration and quality was determined by spectrophotometry (NanoDrop Technologies) and gel electrophoresis, respectively. Total extracted RNA was used for cDNA synthesis. Total RNA was treated with RNAse–free DNAse I during extraction phases. 1 μg of RNA was reverse transcribed to cDNA using Accupower kit (South Korea).
Primer sets of GAPDH and B2M were designed on different exons (intron-spanning) and of β-actin on one exon were designed using Primer Premier V.5 (Premier Biosoft International, Palo Alto, CA, USA), according to the parameters required for the SYBR Green Real-Time PCR [29]. Primer characterization, accession numbers for equine mRNA sequences and length of amplicon are shown in Table 1. The generated cDNAs were analyzed by quantitative real-time PCR. 25 μl reactions were carried out in triplicate in a qRT-PCR cycler (QIAGEN Rotor-Gene 6000 Real-Time Thermal Cycler) using SYBR Green Master Mix (thermo scientific, USA). cDNA samples were diluted 2 times and 2 microlitre of cDNA were used in each reaction along with 200 nM of forward and reverse primers. The cycling conditions were one initial cycle of 94°C for 5 min followed by 40 cycles of 94°C for 30 s, annealing temperature (56.5°C) for 45 s and 72°C for 60 s. The program was ended with a dissociation curve analysis to verify the product and identify the presence of spurious PCR bands or primer dimers. In each experiment, negative control and RT minus sample were used to check template contamination and genomic DNA contamination. Moreover, PCR products were run on 1% agarose gel to confirm specificity of amplification. CT was automatically determined by the Optical System Software (Rotor-Gene Q series software) and these data were exported for further analyses.
CT values of 3 samples in each group (with 3 replicates) were analyzed with t-test using SPSS.16 software. To calculate the average fold change in GAPDH, β-actin and B2M expression in AT- MSCs compared to BM- derived MSCS, the mean CT values was calculated as 2-CT. Then, average fold change was obtained by division of mean of AT- MSCs value to the mean of BM- derived MSCs value [30].
Results
Minimal criteria for identification of isolated mesenchymal stem cells were evaluated [14,31,32]. Isolated cells were plastic adherent with a fibroblast-like phenotype. They showed the pattern of mesenchymal markers expression (CD29, CD44, and CD90) and the lack of expression of MHC-II and CD34 (as hematopoietic marker). In addition, cellular differentiation assays demonstrated the chondrogenic, adipogenic and osteogenic potential of the isolated cells.
All RNA samples had absorbance values at 260 and 280 nm (A260/280) and 260 and 230 nm (A260/230) 2.0–2.2 and 1.8–2.2, respectively. Gel electrophoresis of RNA samples showed two clear bands related to rRNA (28 s and 18 s) along with 5 s band which confirmed the quality of RNA. There was no evidence of contaminating genomic DNA in all runs. Moreover, GAPDH primers amplified fragments 418 bp and 183 bp from DNA whereas amplified only 183 bp fragment from cDNA. No 418 amplicon was observed in gel electrophoresis of PCR products. Likewise, primers were validated by amplification efficiencies (E = 10-1/slope) of 100% ± 10% and the efficiency of reactions was 1.90, 2 and 2.03 for GAPDH, β-actin and B2M respectively (Figure 1).
Fluorescence was measured during each PCR cycle and the amount of fluorescence was proportional to the amount of the PCR product. Actually, CT value is inversely proportional to the total RNA concentration isolated from MSCs, hence CT values for BM-MSCs were greater than AT-MSCs ones. The melting curve analysis showed the specificity of the GAPDH primers (Figure 2). Subsequently, the agarose gel electrophoresis was performed to confirm the size and purity of amplification products in various samples (Figure 3). The mean ± SD of CT values for GAPDH expression in AT-MSCs and BM-MSCs is shown in Table 2. Statistical analysis showed that the expression levels of GAPDH were significantly different between AT- and BM- derived MSCs (p < 0.05). The expression level of GAPDH in AT-derived MSCs compared to BM-derived MSCs was 2.38 times (Figure 4).
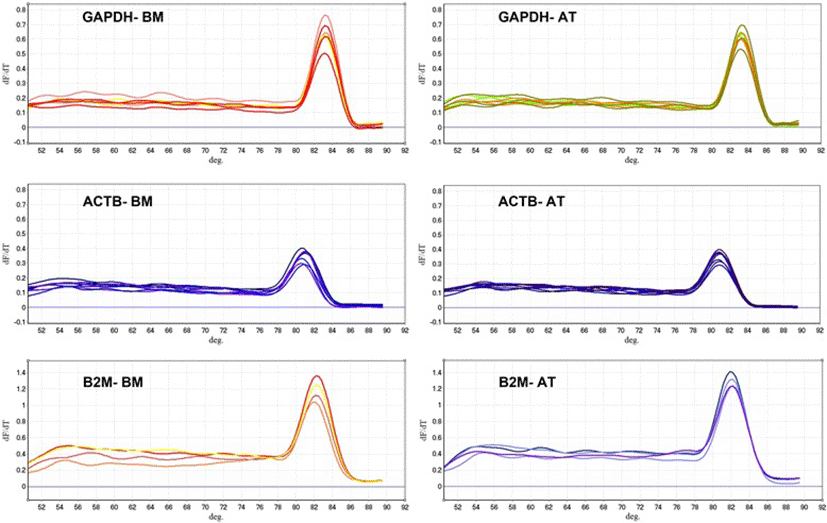
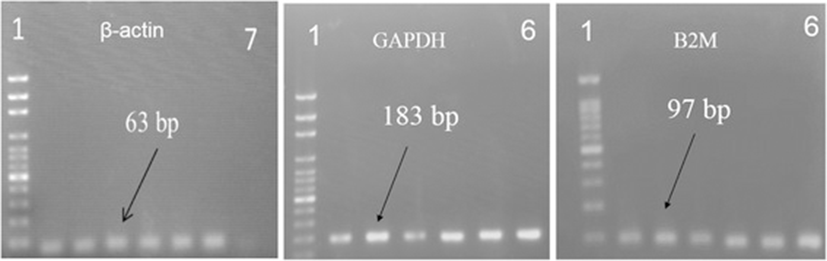
Gene | Case N. | Replicates | BM-MSCs | AT-MSCs | ||
---|---|---|---|---|---|---|
CTmean | SD | CTmean | SD | |||
GAPDH | 3 | 3 | 19.11a | 2.52 | 16.19b | 0.49 |
ACTB | 3 | 3 | 21.97a | 2.21 | 18.05b | 0.70 |
B2M | 3 | 3 | 22.35a | 3.14 | 17.66b | 1.09 |
Letters (a, b) represent significant differences in expression levels of GAPDH (P < 0.05), β-actin (P < 0.001) and B2M (P < 0.006) between BM- and AT-derived MSCs. BM = Bone marrow; AT = Adipose tissue; MSCs = Mesenchymal stem cells.
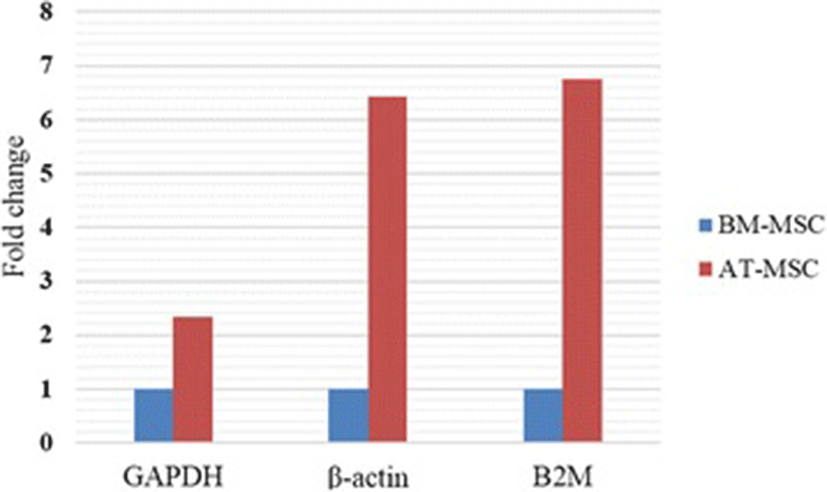
Similar to GAPDH, fluorescence was measured during each PCR cycle for amplifying ACTB amplicon. Again, each run was completed with a melting curve analysis to confirm the specificity of amplification and lack of primer dimmers (Figure 2). Further analysis by agarose gel electrophoresis confirmed the melt curve analysis (Figure 3). The mean ± SD of CT values for expression of β-actin in AT-MSCs and BM-MSCs is shown in Table 2. Statistical analysis showed that differences in expression levels of β-actin in AT- and BM- derived MSCs were significant (p < 0.001). Actually, the expression level of β-actin in AT-derived MSCs compared to BM-derived MSCs was 6.76 times (Figure 4).
cDNA was amplified by qPCR using B2M primers with a melting curve at the end of amplification (Figure 2). Mean ± SD of quantification Cycle (CT) values was determined (Table 2). Statistical analysis indicated significant difference between averages of CT values of two groups (p < 0.006). A single peak in melting curve and lack of nonspecific bands in gel electrophoresis of qPCR products demonstrated that one product was amplified (Figure 3). The expression level of B2M in AT-derived MSCs compared to BM-derived MSCs was 7.76 times (Figure 4).
Discussion
BM-derived MSCs are widely investigated but because of high proliferation and less invasive access of AT-derived MSCs, this source is more attractive [33,34]. It is suggested that the location of MSCs in various tissues results in some differences in their characteristics [6,9,10]. Comparative gene expression analysis is one of the suitable methods to clarify their differences [22]. Absolute and relative methods are two ways for quantification of gene transcripts. To study changes in gene expression levels in terms of quantity, the relative method is used, which present the data of target gene relative to internal control gene (s) [30,35]. GAPDH, β–actin and B2M are frequently used as endogenous controls for quantitative RT-PCR analysis because their expression seems to be consistent at different time points and various experimental manipulations [20,25,36,37]. In contrast, our finding showed that the expression levels of GAPDH and especially β–actin and B2M were significantly different between AT- and BM- derived MSCs. Moreover, the expression levels of both genes in AT-MSCs were much more than BM-MSCs. So, it seems that the location of MSCs cells could affect the expression levels of these genes.
Recently several scientific reports which are working on different research fields about equine and especially equine MSCs have used common housekeeping genes as reference [5,23,24,38,39]. Many studies have tried to introduce a reasonable method to find a reliable reference gene. The stability of reference gene expression is the definition of endogenous control and it is essential to finding the stable one(s) to every experimental condition or tissue location in the body [24,40,41]. Some studies in the field of equine MSCs have used GAPDH, β-actin and B2M as internal control genes without presenting any data regarding the stability of these genes [5,22,23], whereas some studies have confirmed the instability of their expression which is in agreement with our findings [42,43].
Transcription rate of GAPDH, β-actin and B2M can be influenced by a number of factors such as cellular proliferation. The β-actin functions in cellular shape and anchorage [44]. GAPDH, in addition to its role in glycolytic processes, has been involved in membrane transport, microtubule assembly, protein phosphotransferase/kinase reactions, the translational control of gene expression and DNA replication [45]. B2M stimulate cell proliferation in stromal cells so that its over expression and knockdown affect proliferation of MSCs [46]. So, cellular proliferation can induce upregulation of GAPDH, β-actin and B2M gene expression [47]. In agreement with Burk et al. [10], we previously found that the proliferative capacity of AT-MSCs is more than BM-MSCs [31,32] which can explain the greater expression levels of GAPDH, β-actin and B2M in AT-MSCs compared to BM-MSCs.
Conclusion
Although many studies in the field of equine MSCs commonly have used GAPDH, β–actin and B2M as simple internal genes, it seems that their expression levels in MSCs isolated from different tissues is not stable and they could not be considered as suitable reference genes for comparative gene analysis studies. Thus, it is necessary to find and introduce proper reference genes for comparative gene expression analysis studies in equine MSCs isolated from bone marrow and adipose tissue.