INTRODUCTION
Probiotics can be defined as “live microorganisms which, when administered in adequate numbers, confer a health benefit on the host”[1]. Owing to the beneficial effects of probiotics, such as reduction in the incidence and duration of childhood diarrhea, improvement in symptoms of the irritable bowel syndrome, and regulation of intestinal immunity, demands for probiotic-formulated food products are increasing across the world [2,3]. Probiotics have been incorporated in various foods including dairy products (e.g., yogurt, cheese, and ice cream) and non-dairy products (e.g., chocolate, cereals, and juices) and global probiotic market is expected to reach US$46.55 billion by 2020 [4–6]. However, there remain problems on the viability of probiotics when probiotics have been used in foods [4]. To provide their beneficial effects on the host, it is necessary that probiotics survive through the upper gastrointestinal tracts and sufficient numbers of probiotics reach the intestine alive [7]. To reach a sufficient number of viable probiotics to the intestinal epithelium, probiotic foods should contain at least 106–107 CFU/g at the time of consumption [8–10]. However, the number of viable cell in the intestinal epithelium is often low because the number of probiotic can be easily decreased under adverse and harsh conditions during food processing (e.g., heat treatment), storage, and digestion (e.g., acidic pH of stomach) [11]. Therefore, it is important to protect probiotics and keep them alive until reaching the intestine.
Microencapsulation technologies, such as spray-drying, spray-congealing, extrusion, and coacervation, have been used for the protection and delivery of probiotics [4,12,13]. Spray drying is regarded as a low cost process since it is suitable for high-volume production and requires low energy input., Therefore, spray drying has been widely used to encapsulate probiotics in various matrixes and to produce microparticles delivering probiotics [4]. However, high heat temperature (e.g., > 130°C) during spray drying negatively affected the viability of encapsulated probiotics [11,14,15]. Moreover, non-dairy origin delivery materials, such as alginate, gellan-gum, and xanthan, cannot be applied to dairy foods in some countries [11,16]. Therefore, it is necessary to produce an effective delivery system for probiotics using dairy origin delivery materials, such as milk proteins with mild heat treatment.
Rennet is an enzymatic mixture with a protease activity. Chymosin, the major component of rennet, can hydrolyze peptide bond between phenylalanine (residue 105) and methionine (residue106) in κ-casein [17]. The cleavage of κ-casein on the surface of casein micelles results in a decrease in the net negative charge and an increase in their hydrophobicity, which leads to aggregation of casein micelles and forms a gel [11]. Heidebach et al. [11] produced dairy-based probiotic delivery system using the emulsification and rennet-induced gelation of skim milk. This method is relatively simple and suitable for both lab-scale and high volume manufacturing since specialist equipments are not necessary to use. However, in the study of Heidebach et al. [11], very high concentration level (35%, w/w) of skim milk powder was used for the encapsulation of probiotics, which can increase the production cost and lead to form larger particles (~69 μm). Although an increase in the size of delivery system could increase the encapsulation efficiency and viability of probiotics in previous studies [18,19], larger delivery system may negatively affect the textural and sensorial properties of foods [4,18]. Compared with spray drying method performed at high temperature above > 100°C, sub-ambient temperature treatment between 5°C and 25°C was used in rennet-induced gelation method, which could make it advantageous to minimize heat-induced damage for probiotics [20]. Moreover, milk proteins have lower viscosity than highly viscous non-dairy delivery materials including alginate, gellan-gum, and xanthan. Because of high viscosity of those non-dairy delivery materials even at low concentration, the developing non-dairy based gel networks can have the low density, which cannot offer efficient protection for encapsulated materials [11]. Therefore, rennet-induced gelation of milk proteins can be used as an ideal method for the encapsulation of probiotics.
To produce cost-effective probiotic delivery systems, the concentration level of skim milk powder should be reduced (e.g., below 10%). Moreover, to understand how the manufacturing processes can modulate the physicochemical characteristics of probiotic delivery systems including size and zeta potential, it is necessary to study the relationships between the manufacturing variables and physicochemical properties of probiotic delivery systems. In this study, we hypothesized that the manufacturing variables, such as milk protein concentration level and pH, that affect the rennet-induced gelation, may play important roles on the physicochemical properties of probiotic delivery systems, the encapsulation efficiency of probiotics, and viability of probiotics during gastrointestinal digestion.
The objectives of this study were to produce probiotic delivery system using rennet-induced gelation of milk proteins and to study how manufacturing variables, such as skim milk powder concentration level and pH, affect the physicochemical properties of probiotic delivery systems and the viability of encapsulated probiotics during in vitro gastrointestinal digestion.
MATERIALS AND METHODS
Skim milk powder with protein content of 35% (w/w) and rennet (Natural standard plus 290) were purchased from Maeil Dairies (Seoul, Korea) and Chr. Hansen pty. (Bayswater, Melbourne, Australia), respectively. CaCl2, Tween 80, Span 80, NaCl, KCl, NaHCO3, pepsin from porcine gastric mucosa, bile extract porcine, and acridine orange were purchased from Sigma-Aldrich (St. Louis, MO, USA).
Probiotic strain, Lactobacillus rhamnosus GG, was cultured in de Man, Rogosa, and Sharpe (MRS) media (Difco Laboratories, Franklin Lakes, NJ, USA) at 37°C for 18 h. After two subcultures in MRS media, cell suspension was centrifuged at 1,500×g, 4°C for 5 min. The pellet was washed twice with sterile 0.9% (w/v) sodium chloride solution and used for further encapsulation process.
Probiotic delivery systems were produced using the modified rennet-induced gelation of milk proteins described in Heidebach et al. [11]. Skim milk solutions (3, 5, and 10% [w/w]) reconstituted in sterile water were adjusted to pH 5.4 and 6.2 using 1 M HCl and cooled to 5°C. The cell pellet was mixed with 15 mL of skim milk solutions with various concentration levels (3, 5, and 10% [w/w]) and pH (5.4 and 6.2) to obtain probiotic/skim milk mixtures with at least 9.0 log CFU/mL of L. rhamnosus GG. Next, 51.7 μL of rennet was added to 15 mL of probiotic/skim milk mixtures and kept at 5°C for 60 min. Seventy-five microliters of 1 M CaCl2 were added to 15 mL of probiotic/skim milk/rennet mixtures. The final concentration of CaCl2 in the mixture was 5 mM. To form oil-in-water (O/W) emulsions, 15 mL of those mixtures were added to 160 g of corn oil containing 5% (w/w) span 80 and then homogenized at 8,000 rpm for 5 min using a probe-type homogenizer (Daihan scientific, Wonju, Korea). To prevent rennet-induced gelation of milk proteins, temperature was kept at 5°C during homogenization. After the formation of O/W emulsions, the temperature of emulsions was controlled to 25°C and kept for 10 min to induce the rennet-induced gelation of milk proteins. To obtain probiotic delivery systems, O/W emulsions were centrifuged at 15,000 ×g, 4°C for 1 min and then oils at top layer were removed. After washing 3 times with distilled water to remove residual oils, probiotic delivery systems were collected and freeze dried.
Formation and morphological properties of probiotic delivery systems were determined using a confocal laser scanning microscope (CLSM, Olympus FV-1000, Tokyo, Japan). To monitor probiotic delivery systems, acridine orange, a fluorescent dye, was used to stain milk proteins. Prior to the production of delivery systems, 90 μL of 0.2% (w/w) acridine orange was added to 15 mL of 3, 5, and 10% (w/v) skim milk solutions and adjusted to pH 5.4 and 6.2 with 1 M HCl followed by cooling to 5°C. Probiotic delivery systems were manufactured according to methods described above. After freeze drying, 0.15 g of probiotic delivery systems were placed on a concave confocal microscope slide. The excitation and emission wavelengths were set at 488 and 526 nm, respectively.
Particle size analyzer (1090LD shape, CILAS, Orleans, France) was used to measure the particle size (volume-mean diameter, D43) and size distribution (span value) of probiotic delivery systems. Prior to measure the size, 0.15 g of freeze-dried probiotic delivery systems were dispersed in 45 mL of 10% (w/w) tween 80 solution followed by sonication at 50 W for 3 min. Span value was calculated by following equation [21].
where D (0.9), D (0.1), and D (0.5) are particle diameter at cumulative size of 90%, 10%, and 50%, respectively. A low span value indicates uniformity in size distribution.
The encapsulation efficiency of probiotics in milk-protein based delivery systems was evaluated by counting viable cells in delivery systems using a standard plate method on MRS agar. Freeze-dried probiotic delivery systems were dispersed in 10% tween 80 solution and the number of viable cells was determined. To determine the number of viable cells in milk protein-based delivery systems, reconstituted delivery systems were enzymatically hydrolyzed using the modified method of Heidebach et al. [11]. Reconstituted delivery systems were diluted 10 times with Protease N “Amano” (0.0024 g/10 mL) and kept at 40°C for 30 min with constant agitation at 150 rpm using a shaking water bath. After reaction with Protease N “Amano”, viable cells were enumerated using a standard plate method on MRS agar. Encapsulation efficiency was calculated by following equation [22].
where N0 is the initial number of L. rhamnosus GG added in the preparation process and N is the total number of L. rhamnosus GG encapsulated in probiotic delivery systems.
The viability of encapsulated L. rhamnosus GG under simulated gastric and intestinal conditions was evaluated by the modified method of Chávarri et al. [23] and García-Sartal et al. [24]. To prepare simulated gastric juice (SGJ), pepsin (0.3%, w/v) was dissolved in 0.9% (w/v) NaCl solution and adjusted to pH 2.0. Simulated intestinal juice (SIJ) was prepared with 0.65% (w/v) NaCl, 0.0835% (w/v) KCl, 0.022% (w/v) NaHCO3, and 0.3% (w/v) bile extraction porcine and then was adjusted to pH 7.5. SGJ and SIJ were filtered with a 0.45 μm syringe filter. Free and encapsulated probiotics (L. rhamnosus GG) were 10-fold diluted with SGJ and SIJ and then incubated at 37°C for 120 min with constant agitation at 150 rpm using a shaking water bath (Daihan scientific). After incubation, SGJ was diluted 10 times with 0.05 M sodium phosphate buffer (pH 7.0) for inactivation of pepsin. To determine the number of viable cells in milk protein-based delivery systems, delivery systems were enzymatically hydrolyzed using the modified method of Heidebach et al. [11] described earlier.
All data were expressed as a mean of three replicates. The impacts of manufacturing variables, such as skim milk concentration level and pH, on the particle size and size distribution of probiotic delivery systems were determined by one-way analysis of variance (ANOVA) with Fisher’s significant differences test with statistical significance of p < 0.05. Repeated-measures ANOVA was used to determine the effects of manufacturing variables, incubation time, and their interactions on the survival of L. rhamnosus GG in simulated gastrointestinal conditions. The statistical analysis system (Version 9.1, SAS Institute, Cary, NC, USA) was used to perform ANOVA.
RESULTS AND DISCUSSION
Modified rennet-induced gelation method [11] was used to manufacture milk protein-based probiotic delivery systems. The formation and morphological properties of probiotic delivery systems were determined using CLSM (Figs. 1 and 2). Round shaped particles with a size ranging from 100 to 200 μm were observed indicating the successful development of probiotic delivery systems. An increase in the size of probiotic delivery systems was observed with an increase in the skim milk powder concentration level from 3 to 10% (w/w) (Fig. 1) and in pH from 5.4 to 6.2 (Fig. 2).

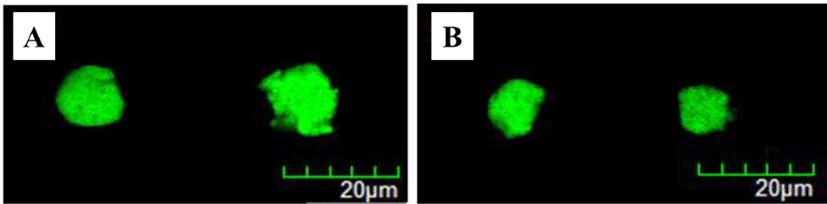
Impacts of skim milk powder concentration level and pH on the size of probiotic delivery systems were assessed by using particle size analyzer (Fig. 3). As skim milk powder concentration level was increased from 3 to 10% (w/w), the size of probiotic delivery system was significantly (p < 0.05) increased from 27.5 to 44.4 μm (Fig. 3A). The cleavage of negatively charged κ-casein molecule existed on the surface of casein micelles by rennet result in a reduction in electrostatic repulsions between casein micelles. It can lead to an increase in the aggregation of casein micelles resulting in the formation of gels [11]. Since more casein micelles as building blocks may participate in the production of probiotic delivery system at higher skim milk powder concentration level, their intermolecular associations could be increased with an increase in skim milk powder concentration level, which may lead to the formation of thicker and bigger protein network. This may result in an increase in the size of milk protein-based probiotic delivery systems.
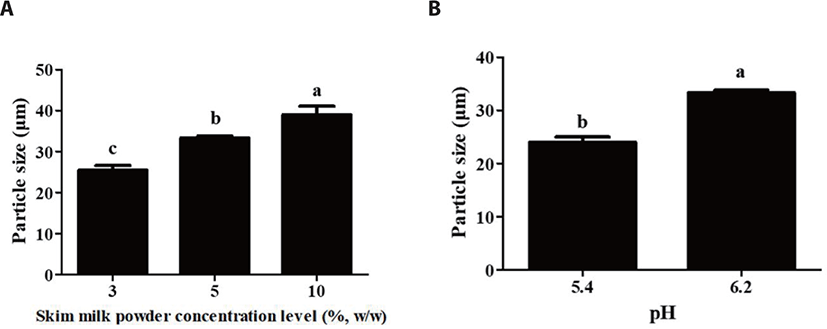
A decrease in pH from 6.2 to 5.4 resulted in a significant (p < 0.05) decrease in the size of probiotic delivery systems (Fig. 3B). Because the isoelectric pH of casein micelles is ~4.6, a decrease in pH from 6.2 to 5.4 lead to a decrease in the net negative charges of casein micelle and therefore hydrophobic interactions between casein micelles could be increased [11,17]. An increase in the hydrophobic interactions between casein micelles may lead to an increase in the intramolecular associations of protein gel network, which may result in the shrinkage of gel network and formation of more compact and small particles at pH 5.4 [11,25].
The size distribution of probiotic delivery systems was shown in Fig. 4. Span value is a statistical parameter that can be useful to evaluate the distribution of particle size. Smaller the span value indicates narrower size distribution (homogeneous) [21,26]. In all conditions, span values of probiotic delivery systems were ranged from 1.5 to 3.0 indicating that probiotic delivery systems had narrow size distribution and formation of homogeneous particles [27].
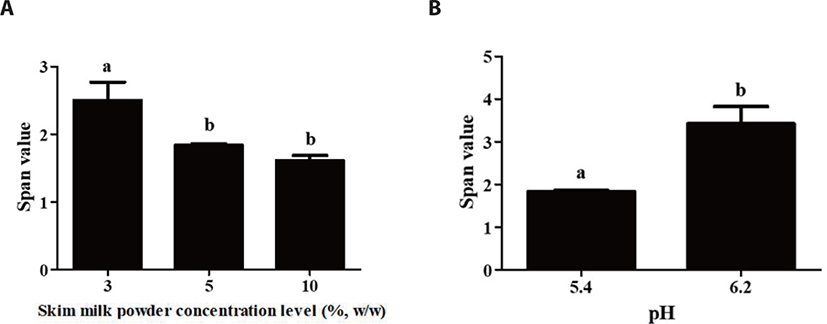
Fig. 5 shows the effects of skim milk powder concentration level and pH on the encapsulation efficiency of probiotics. An increase in the skim milk powder concentration level from 3 to 10% (w/w) resulted in a significant (p<0.05) increase in the encapsulation efficiency of probiotics from 76.0% to 88.5% (Fig. 5A). An increase in skim milk powder concentration level may lead to the formation of bigger and thicker milk protein-based delivery systems (Fig. 3A). Therefore, it could provide more effective barriers to encapsulated probiotics resulting in an increase in the encapsulation efficiency of probiotics. Similar results were reported by Sheu et al. [18] and Lee and Heo [28]. They reported that more probiotics were encapsulated in bigger delivery systems.
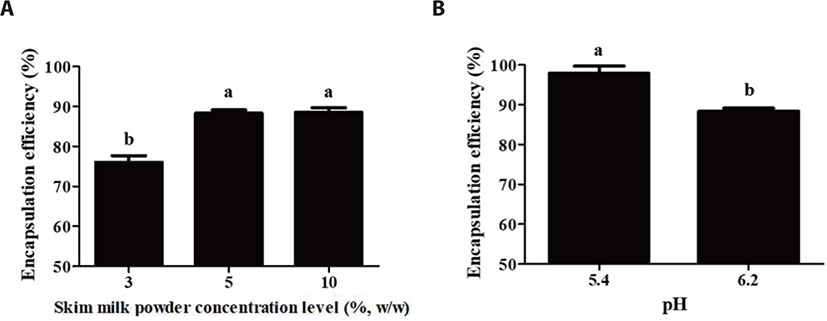
Although the particle size of probiotic delivery systems was decreased as pH was decreased from 6.2 to 5.4, encapsulation efficiency of probiotics was significantly (p<0.05) increased from 88.3 to 97.8% with a decrease in pH (Fig. 5B). Because same protein concentration level (5%, w/w) was used to manufacture those probiotic delivery systems at different pH, an increase in encapsulation efficiency may be due to increased intramolecular associations between protein molecules in rennet-induced milk protein gel networks. It is known that chymosin has the optimum pH for the hydrolysis of κ-casein, which is 5.1–5.3 [29,30]. Imafidon and Farkye [31] also reported that the κ-casein cleavage by chymosin was greatest at pH 5.3-5.6. These results indicate that a decrease in pH from 6.2 to 5.4 could lead to an increase in the activity of chymosin on the hydrolysis of κ-casein. Since an increase in the hydrolysis of κ-casein could contribute to a decrease in electrostatic repulsions and an increase in hydrophobic attractions between casein micelles, more compact and denser protein networks could be formed at lower pH resulting in an increase in the encapsulation efficiency of probiotics [11,32,33].
Impacts of encapsulation in milk protein-based delivery systems on the viability of probiotic stain, L. rhamnosus GG, during incubation under gastrointestinal conditions were presented in Table 1. Repeated measures ANOVA exhibited that encapsulation in milk protein-based delivery system (treat, p < 0.0001), incubation time (time, p = 0.0005), and their interaction (p < 0.0001) in SGJ had a significant effect on the viability of L. rhamnosus GG (Table 1). The number of viable cells was decreased during incubation in SGJ. A decrease in the viability of probiotics can be attributed to the high acidity of gastric juice under gastric conditions. When L. rhamnosus GG was encapsulated in milk protein-based delivery system, overall mean viable cell number was significantly (p = 0.0005) increased from 7.35 to 7.88 CFU/mL. It implies that encapsulation in milk protein-based delivery system is a useful method to enhance the viability of L. rhamnosus GG in gastric condition. When free and encapsulated L. rhamnosus GG were exposed to SIJ, the repeated measures ANOVA results presented that encapsulation (treat, p = 0.0934), incubation time (time, p = 0.8153), and their interaction (p = 0.7306) were not significant indicating that bile salts and intestinal pH did not affect the viability of L. rhamnosus GG was not affected under intestinal juice condition, such as bile salts and intestinal pH.
Table 2 shows the impacts of manufacturing variables, such as skim milk powder concentration level and pH on the viability of encapsulated probiotics, L. rhamnosus GG, in simulated gastrointestinal conditions. Repeated measures ANOVA revealed significant effects on the skim milk powder concentration level (treat, p = 0.0001), incubation time (p < 0.0001), and their interaction (p < 0.0001) on the viability of L. rhamnosus GG in SGJ while no significant effects were observed in SIJ (Table 2). As skim milk powder concentration level was increased from 3 to 10% (w/w), the overall viability of L. rhamnosus GG in SGJ was increased from 7.34 to 7.69 CFU/mL indicating that skim milk powder concentration level can be a key factor to enhance the viability of probiotics in gastric condition. An increase in protein content by increasing skim milk powder concentration level may enhance the intermolecular associations between casein micelles resulting in the production of thicker protein networks. Those thicker protein gel networks could reduce the diffusion of gastric juice with very high acidity into the probiotic delivery systems [34]. Therefore, delivery systems prepared with higher milk protein concentration level can provide better protections for probiotics in harsh gastric conditions compared with delivery systems treated with lower milk protein concentration level. Similar results were reported by Shi et al. [34] who presented that probiotic delivery systems produced with higher milk concentration could provide better protections for L. bulgaricus in SGJ.
2) Probiotics (Lactobacillus rhamnosus GG) were encapsulated in milk protein-based delivery system prepared with various skim milk powder concentration levels (3, 5, and 10% [w/w]) at pH 6.2.
In repeated measures ANOVA, a significant effect of pH (treat, p < 0.0001), incubation time (p < 0.0001), and their interaction (p = 0.0003) on the viability of of L. rhamnosus GG in SGJ was observed while there were no significant effects observed in SIJ (Table 2). Overall viability of L. rhamnosus GG in SGJ was increased from 7.88 to 8.33 CFU/mL as pH was decreased from 6.2 to 5.4. As we described earlier, a decrease in pH 6.2 to 5.4 may enhance hydrophobic attractions between casein micelles forming more compact and denser protein gel networks. The production of denser protein gel networks could reduce acid diffusions into milk protein-based delivery systems in SGJ, which may contribute to higher viability of L. rhamnosus encapsulated in milk protein-based delivery system prepared at pH 5.4.
In conclusion, probiotic strain, L. rhamnosus GG, was successfully encapsulated in milk protein-based delivery systems using a modified rennet-induced gelation method. Skim milk powder concentration level and pH were major manufacturing variables affecting the physicochemical properties of milk protein-based probiotic delivery systems, such as a particle size, size distribution, encapsulation efficiency, and viability of probiotics in simulated gastrointestinal tract. It can be attributed to intra- and intermolecular associations between milk protein molecules during rennet-induced gelation. Milk protein-based delivery systems can be used for enhancing the viability of probiotics and these food-grade probiotic delivery systems can be useful to apply probiotics to various foods.